How Microbes Can Help Feed the World
Authors
Ann Reid and Shannon E. Greene.ABSTRACT
According to the United Nations World Food Program, more than 870 million of the world's people are malnourished. Many of the hungry are children. In the developing world, malnutrition contributes to the death of 2.6 million children each year and one of six children is underweight. At the same time that more food is desperately needed, arable land and important resources like fertilizer and water are limited, and salinization and climate change limit the suitability of much land for agricultural production. Feeding a global population that is projected to reach 9 billion by 2050 will require that agricultural yields increase by 70–100%.
Yields of any given crop vary widely from place to place, even in the same region. Those who study global agricultural trends speak of the ‘yield gap’, which is the difference between the best observed yield and results elsewhere. Theoretically, if that gap could be closed — if all farmers could achieve the highest attainable yield — worldwide crop production would rise by 45–70%. Yield gaps can often be explained by inadequate fertilizer or water, or by losses to pests or disease, but vast increases in use of fertilizers, water, and pesticides are not only economically impractical, but would have many negative environmental consequences. Scaling up current high-input agricultural systems is simply not feasible.
But what if the yield gap could be closed in another way, if yield could be increased with dramatically fewer chemical inputs? Producing more food with fewer resources may seem too good to be true, but the world's farmers have trillions of potential partners that can help achieve that ambitious goal. Those partners are microbes.
In December 2012, the American Academy of Microbiology convened 26 experts in plant-microbe interactions to discuss how microbes could help feed the world. The group included scientific experts on plant-microbe interactions of many different kinds: development experts who seek to introduce new technologies and practices in developing countries, representatives of companies that develop microbial products, and policy-makers from the public and non-profit sectors. Together, over the course of two days, the group explored the many ways that microbes and plants interact and developed a vision for how those interactions could be employed to boost agricultural productivity in an environmentally and economically responsible way. Their vision is described in this report. The Academy would like to thank the National Science Foundation and the United States Department of Agriculture's National Institute for Food and Agriculture for supporting this colloquium.
Front Matter
The American Academy of Microbiology is the honorific branch of the American Society for Microbiology, a non-profit scientific society with almost 40,000 members. Fellows of the AAM have been elected by their peers in The American Academy of Microbiology is the honorific branch of the American Society for Microbiology, a non-profit scientific society with almost 40,000 members. Fellows of the AAM have been elected by their peers in recognition of their outstanding contributions to the field of microbiology. Through its colloquium program, the AAM draws on the expertise of these fellows to address critical issues in microbiology. recognition of their outstanding contributions to the field of microbiology. Through its colloquium program, the AAM draws on the expertise of these fellows to address critical issues in microbiology.
This report is based on the deliberations of experts who gathered for two days to discuss a series of questions about how plant microbe interactions could be employed to boost agricultural productivity in an environmentally and economically responsible way.
The report has been reviewed by all participants, and every effort has been made to ensure that the information is accurate and complete. The contents reflect the views of the participants and are not intended to reflect official positions of the American Academy of Microbiology or the American Society for Microbiology.
Contents of the report may be distributed further so long as the authorship of the AAM is acknowledged and this disclaimer is included.
The American Academy of Microbiology is grateful for the generosity of the following organizations for their support of this project:
- ▪ National Science Foundation
- ▪ United States Department of Agriculture's National Institute for Food and Agriculture
COLLOQUIUM PARTICIPANTS
STEERING COMMITTEE
CHAIR
Ian R. Sanders, Ph.D. University of Lausanne
Ann Lichens-Park, Ph.D. National Institute of Food and Agriculture of the U.S. Department of Agriculture
Marilyn J. Roossinck, Ph.D. Pennsylvania State University
Linda S. Thomashow, Ph.D. Washington State University
Ryland Young, III, Ph.D. Texas A&M University
PARTICIPANTS
Harsh Bais, Ph.D. University of Delaware
Gwyn Beattie, Ph.D. Iowa State University
Michael P. Doyle, Ph.D. University of Georgia
Ken Giller, Ph.D. Wageningen University
Bernard R. Glick, Ph.D. University of Waterloo
Charles Godfray, Ph.D., FRS, CBE University of Oxford
Gabriel Iturriaga, Ph.D. Aguirre Innovación SC.
Linda Kinkel, Ph.D. University of Minnesota
Steven E. Lindow, Ph.D. University of California, Berkeley
Joyce Loper, Ph.D. USDA Agricultural Research Service and Oregon State University
Matteo Lorito, Ph.D. University of Naples
Mary E. Maxon, Ph.D. Lawrence Berkeley National Laboratory
Jos Raaijmakers, Ph.D. Wageningen University
Rusty Rodriguez, Ph.D. Symbiogenics and University of Washington
Alia Rodriguez Villate, Ph.D. Universidad Nacional de Colombia
Daniel Segrè, Ph.D. Boston University
Gary A. Strobel, Ph.D. Montana State University
Eric Triplett, Ph.D. University of Florida
Prem Warrior, Ph.D. Bill & Melinda Gates Foundation
Peter Young, Ph.D. University of York
Christin Zachow, Dr. Dipl. Biol. Austrian Centre of Industrial Biotechnology
AMERICAN ACADEMY OF MICROBIOLOGY STAFF
Ann Reid Director
Leah Gibbons Colloquium and Public Outreach Program Assistant
Shannon E. Greene, Ph.D. Colloquium Fellow
Michael Ingerson-Mahar, Ph.D. Colloquium Fellow
PREFACE
According to the United Nations World Food Program, more than 870 million of the world's people are malnourished. Many of the hungry are children. In the developing world, malnutrition contributes to the death of 2.6 million children each year and one of six children is underweight. At the same time that more food is desperately needed, arable land and important resources like fertilizer and water are limited, and salinization and climate change limit the suitability of much land for agricultural production. Feeding a global population that is projected to reach 9 billion by 2050 will require that agricultural yields increase by 70–100%.
Yields of any given crop vary widely from place to place, even in the same region. Those who study global agricultural trends speak of the ‘yield gap’, which is the difference between the best observed yield and results elsewhere. Theoretically, if that gap could be closed — if all farmers could achieve the highest attainable yield — worldwide crop production would rise by 45–70%. Yield gaps can often be explained by inadequate fertilizer or water, or by losses to pests or disease, but vast increases in use of fertilizers, water, and pesticides are not only economically impractical, but would have many negative environmental consequences. Scaling up current high-input agricultural systems is simply not feasible.
PRODUCING MORE FOOD WITH FEWER RESOURCES MAY SEEM TOO GOOD TO BE TRUE, BUT THE WORLD'S FARMERS HAVE TRILLIONS OF POTENTIAL PARTNERS THAT CAN HELP ACHIEVE THAT AMBITIOUS GOAL. THOSE PARTNERS ARE MICROBES.
But what if the yield gap could be closed in another way, if yield could be increased with dramatically fewer chemical inputs? Producing more food with fewer resources may seem too good to be true, but the world's farmers have trillions of potential partners that can help achieve that ambitious goal. Those partners are microbes.
In December 2012, the American Academy of Microbiology convened 26 experts in plant-microbe interactions to discuss how microbes could help feed the world. The group included scientific experts on plant-microbe interactions of many different kinds: development experts who seek to introduce new technologies and practices in developing countries, representatives of companies that develop microbial products, and policy-makers from the public and non-profit sectors. Together, over the course of two days, the group explored the many ways that microbes and plants interact and developed a vision for how those interactions could be employed to boost agricultural productivity in an environmentally and economically responsible way. Their vision is described in this report. The Academy would like to thank the National Science Foundation and the United States Department of Agriculture's National Institute for Food and Agriculture for supporting this colloquium.
INTRODUCTION
Microbes already help feed the world. In fact, without microbes there would be no plants or animals, as all life on Earth is dependent on microbes to provide many essential services. Increasingly, biologists are recognizing that all multicellular organisms from sponges to termites to humans are dependent on intimate, evolutionarily-ancient relationships with many different kinds of microbes. For plants, vulnerable as they are to changes in their immediate environment, the services provided by microbes are critical. In their natural, unmanaged environments, all plants are supported by a vast, invisible world of bacteria, viruses, and fungi that live in and around their roots, stems, leaves, seeds, pollen, fruits, and flowers. Additional complex communities of microbes live in and on the insects, birds, invertebrates, and other animals that interact with plants and with each other. These interlocking, interdependent communities have deep evolutionary roots, reaching all the way back to the origin of multicellular organisms and the emergence of land plants and animals. Their diversity and capabilities are nothing short of astounding.
MICROBES AND PLANTS ARE INTIMATE PARTNERS IN VIRTUALLY EVERY LIFE PROCESS.
Microbes support plant health by increasing the availability of nutrients, enhancing plant root growth, neutralizing toxic compounds in the soil, making plants more resistant to disease, heat, flooding, and drought, and deterring pathogens and predators. Microbes and plants are intimate partners in virtually every life process.

How is it that plants can grow in the scalding soil around Yellowstone's hot springs? How do plants survive in deserts? How do they survive in environments where they may spend weeks underwater due to seasonal flooding, or where the soil is salty, contaminated with heavy metals, or poor in nutrients? The answer, in every case, is because of the microbial communities that help the plants overcome these environmental challenges.
Some of the ways microbes contribute to plant health have been known for a long time, and new ones regularly continue to be discovered. Recently, a realization has begun to take hold that these relationships are more vast and important than previously recognized. In the past 20 years or so, our ability to study these complicated communities and unravel the detailed mechanisms by which the members interact has improved enormously.
THE INTIMATE RELATIONSHIP BETWEEN MICROBES AND AGRICULTURE
OPTIMIZING THE MICROBIAL COMMUNITIES OF PLANTS OFFERS AN ENTIRELY NEW APPROACH TO ENHANCING PRODUCTIVITY.
Increasing our knowledge of plant-microbe interactions has deep implications for agriculture. When humans domesticated plants and animals thousands of years ago, they did so without any knowledge of the local microbial communities that were essential to the health and productivity of those plants and animals. Multiple strains of wheat, corn, rice, and other crops have been planted around the world in environments where the local microbial communities differ from those where the plants originated, and where conditions are such that the plant might need new microbial partners to grow best. Historically, traditional plant breeding and genetic engineering, irrigation, and chemical treatments like fertilizers and pesticides have all been used to enhance crop productivity as crops spread to new areas. Optimizing the microbial communities of plants offers an entirely new approach to enhancing productivity. Indeed, such an approach is the opposite of past management strategies that targeted microbes in the mistaken belief that they all cause disease.
Some beneficial microbes have been known for a long time; the microbes known as Rhizobia that inhabit the roots of leguminous plants and provide them with usable nitrogen, were described by Martinus Beijerinck in 1888. (Beijerinck, a much less famous but no less distinguished contemporary of Koch and Pasteur, also discovered sulfate-reducing bacteria and viruses.) Other observations, for example, that certain plant diseases seemed to be rarer in some fields than others, led to research that showed that the “disease suppression” was due to living organisms. Even before the responsible organisms were identified, farmers could move soil from one field to another to take advantage of its protective abilities.

Figure
MARTINUS BEIJERNICK
In the last fifty years, a great deal has been learned about a few of the microbes that live in, on, and near plants. In the last ten years, scientists have made tremendous progress in describing the incredible diversity of this microbial world, and have begun to dissect the elaborate networks of communication and cooperation among plants, insects, invertebrates, grazers, and microbes, and determine the precise molecular mechanisms of such interactions. Commercial applications of these discoveries are already being used to support plant growth, health, and productivity. However, the as-yet-unrealized benefits of understanding how microbes support plant vitality could be immense. Simply recognizing that our major crop plants have been bred and cultivated without attention to their ancestral microbial partners is in itself potentially revolutionary in its implications. The possibility exists of an array of agricultural practices and products that could increase the productivity of any crop, in any environment, in an economically viable and ecologically responsible manner.
This report explores some of the many roles that microbes play in plant health and how plant-microbe interactions could be employed to improve crop productivity. It ends with grand challenges, and a description of the research and development that will be required to meet those challenges. The ubiquity and diversity of plant-microbe interactions can seem almost overwhelming, especially since science has barely scratched the surface of these fundamental biological partnerships. The potential for societal gain is so high, however, that this crucial field of study will richly reward additional attention and investment.
WHY DO PLANTS NEED MICROBES?
VIRTUALLY EVERY ASPECT OF PLANT BIOLOGY IS AFFECTED BY INTERACTIONS WITH MICROBES.
When we think about a corn plant or an apple tree, it seems straightforward to list the factors that affect their health and productivity: sunlight, water, reasonable temperatures, and fertile soil. But in nature, these factors are rarely found altogether at all times. How then do plants survive when one or another of these factors is absent or limited for some period of time? The answer is that there is another, mostly invisible, but crucially important, ingredient involved in the well-being of every plant. Virtually every aspect of plant biology is affected by interactions with microbes. Given fluctuations in the natural environment, plants literally could not survive without microbes and therefore all plants form a multitude of relationships with many different kinds of microbes. Some of these relationships are essential and enduring, others are transient or needed only in certain circumstances. Most of them are probably evolutionarily ancient, but new partnerships are evolving constantly as the environment places selective pressure on communities of plants and microbes.
The relationships between plants and microbes date back to the origin of plants. The early evolution of plants took place in an extraordinarily diverse microbial world; bacteria, archaea and viruses had been evolving for billions of years and occupied every conceivable environmental niche. A new partnership between eukaryotic cells and cyanobacteria led to the acquisition of chloroplasts and set the stage for the evolution of plants. This transformative evolutionary event allowed plants to break into the crowded microbial world by co-opting the ability of cyanobacteria to turn sunlight and carbon dioxide (CO2) into easily digestible sugars, and using that ability to drive the evolution of a myriad of multicellular forms that could carry out that reaction at much larger scales and store the resultant fixed carbon for the next generation. While chloroplasts may be the most ancient evidence of the intertwined evolutionary trajectories of plants and microbes, there are many examples of other long-standing evolutionary relationships, of which the mutualistic symbiosis between leguminous plants and nitrogen-fixing rhizobial bacteria is probably the most familiar, though by no means the most common.
PLANT-MICROBE INTERACTIONS ARE NOT LIMITED TO BACTERIA. PLANTS HAVE INTIMATE, LONG-STANDING RELATIONSHIPS WITH VIRUSES AND FUNGI AS WELL.
Plant-microbe interactions are not limited to bacteria. Plants have intimate, longstanding relationships with viruses and fungi as well. How did all these relationships come about? The ability to carry out photosynthesis and store that energy in the form of leaves, fruits, tubers, and roots makes plants a highly attractive food source. How do they manage to avoid being consumed, especially since they cannot run away? Furthermore, light and CO2 are not the only inputs plants need; they also require a regular supply of water, as well as nutrients like nitrogen and phosphorus, and trace minerals like iron. How do they acquire those inputs, without being able to travel to find them? And most plants reproduce sexually — how do they go about finding a partner when they cannot move?
Many answers to these questions are found in the genomes of plants themselves; the multitude of flower shapes, odors, and colors that attract pollinators, the leaf shapes and arrangements of branches that maximize surface area for photo-synthesis, the stomata that open and close to regulate gas and water exchange — all of these functions are encoded in the genome of the plant itself. But many other answers to these questions are found in the partnerships that plants have made with microbes of many different kinds. A multitude of mutually beneficial relationships in which the plant provides shelter or sugars and the microbe provides nutrients or protection from pathogens have evolved over time.
The number of recognized microbe-plant interactions is large and growing all the time, and they can be classified in several different ways. Most importantly, while we are becoming increasingly aware of mutually beneficial relationships, interactions between plants and microbes can also be neutral or harmful. The conceptual shift that needs to take hold is that plant health is intimately tied up with a complex and largely invisible ecosystem in which literally thousands of species are competing or cooperating in response to constantly changing environmental conditions.
A WHO'S WHO OF MICROBIAL PLANT PARTNERS
There are three major groups of microbes associated with plants. The kinds of services they provide to plants overlap in many ways and may be provided by a single type of microbe or by more than one of them working together. The relationships among these microbes themselves may also be beneficial, neutral, or antagonistic.
BACTERIA

Bacteria are fantastically abundant; there are up to 1010 bacterial cells per gram of soil in and around plant roots, a region known as the rhizosphere. Bacteria are also tremendously genetically diverse — that same gram of soil may contain up to 10,000 different species of bacteria. In addition to the soil, many other bacterial species occupy a variety of niches on and within the aboveground parts of plants. Among the many services that bacteria can provide are: acquisition of nutrients and minerals; production of antibiotics to deter pathogens and toxins to deter pests; and production of hormones and other compounds to spur growth, stimulate the immune system, and modulate responses to stress. Bacteria also participate in complex communities in which one member may secrete a signal that attracts beneficial organisms, another may ward off pathogens, and still another may produce a substrate upon which other beneficial microbes feed. Together these communities may form dense structures called biofilms that have distinct members and properties, serving, for example, as communication and transportation networks.
FUNGI

It has recently become clear that the number and diversity of fungi associated with plants is more vast than previously appreciated. There are 105 to 107 fungal cells per gram of soil, and that reflects only part of the story because fungi live not only on or around plants, but also within them. Every plant that has been sampled has one or many endophytic fungi. The fungi have been found in association with most plant tissues, living between and within plant cells, and forming extensive networks of which only some functions are known. Arbuscular mycorrhizae (AM) are found in association with the roots of 80% of land plants (another 10% of plants, mostly trees, associate with another kind of fungus, discussed below). These fungal symbionts form long filaments, or hyphae, that act as extensions of the plant's roots and are critical in the acquisition of nutrients, minerals, and water. Endophytic fungi can also be found in above-ground plant tissue. For example, Clavicipitaceae endophytes form intercellular networks within their partner grasses where they produce toxins that ward off insect pests and grazing animals. An emerging consensus asserts that many plants in extreme environments survive only because they have formed partnerships with fungi that provide essential tools to survive heat, drought, salt, heavy metal, and other stresses.
Not all beneficial fungi are endophytic. Many trees have fungal symbionts called ectomycorrhizal fungi that form dense mats around tree roots. These fungi are thought to carry out many of the same functions as abuscular mycorrhizal fungi: protection against pathogens and acquisition of water and nutrients.
VIRUSES
Of the many microbes upon which plants are increasingly recognized as being dependent, perhaps viruses are the most surprising. Viruses are far and away the most numerous biological entities on Earth. Every living organism can be infected by at least one and usually many viruses, and most organisms are infected by a diverse and unexplored collection of viruses. However, viruses are rarely considered outside of their role as pathogens. This is understandable; viruses were discovered because of their role in disease. The idea that viruses might be contributing to the healthy state has been slow to emerge. Gradually, though, examples are accumulating of situations where viruses are not only beneficial, but may be essential to some plants.
The ability of viruses to affect plant phenotype is well-known to plant breeders, as it is a virus that produces the streak or flame-like markings in tulip flowers. During the tulip breeding craze in 17th century Holland, breeders knew that they could introduce the streaks by grafting bulbs of striped and plain tulips together. They also knew that the striped varieties gradually declined in health and could not be maintained permanently. But it was only in the 1920's that the “tulip breaking virus” was identified as the reason behind striping. The first virus identified (in 1898) was tobacco mosaic virus, and there are now about 1000 classified plant viral pathogens. But plant viruses have largely been identified because they are causing damage; the idea that some plants may be thriving because of viruses they carry is a relatively new one.
The few examples that have been identified to date suggest that viruses are often essential partners to plants growing in extreme environments. Studies have shown that many plant viruses that cause disease under benign conditions act to improve tolerance when plants are drought stressed. In some cases viral infection may be essential for survival under stressed conditions; the panic grass that grows in the hot soils of Yellowstone National Park can only survive if it carries a certain fungus that is itself infected by a virus. Many plants and fungi carry persistent viruses that are passaged vertically for indefinite time periods. These may serve as cytoplasmic epigenetic elements, providing novel genetic information beyond the plant and fungal genomes.
THE ECOLOGICAL APPROACH THAT PLANTS THEMSELVES HAVE USED TO OVERCOME ENVIRONMENTAL CHALLENGES HAS BEEN ALMOST COMPLETELY IGNORED.
The theme that carries through all of these examples of beneficial plant-microbe interactions is that over billions of years plants have formed partnerships with a variety of microbes to allow them to survive when environmental conditions are not ideal. When levels of nutrients or water are inadequate, when temperatures are too high or too low, when competitors, predators, or pathogens threaten, often plants have been able to draw on microbial partners to overcome these challenges. The implications of this realization for agriculture are profound. For millennia, farming practices have sought to provide ideal conditions for plant growth, using mechanical (irrigation), chemical (fertilizer) and genetic (breeding) approaches to provide an environment that maximizes plant productivity. The ecological approach that plants themselves have used to overcome environmental challenges has been almost completely ignored.
WHAT KINDS OF SERVICES CAN MICROBES PROVIDE?
The aspects of plant biology that microbes affect are comprehensive and take many forms. At any given time, microbial activities may be more or less important for each of these functions, but particularly under conditions of scarcity or stress, microbes are likely to be crucial to plant survival via one or more of the mechanisms described below.
ACQUISITION OF NUTRIENTS
In addition to water, plants need nitrogen, phosphorous, potassium, sulfur, iron and other trace elements. Acquiring them is an ongoing challenge for plants and microbes are active participants in most of these processes. Indeed, bacteria are the only known organisms that can transform gaseous nitrogen into an organic form, ammonia, that can be used by plants. Prior to the development of industrial means to fix nitrogen, plants were entirely dependent on microbes for usable nitrogen.
Plants can only absorb inorganic P (Pi). Both fungi and bacteria can help plants obtain adequate phosphorous. Bacteria produce organic acids that bind Pi and extracellular phosphatases that release Pi from organophosphates and increase its availability to the plant. The hyphae produced by mycorrhizal fungi reach out beyond the zone exploited by plant roots to obtain phosphorus and transport it back to the plant; indeed cassava plants inoculated with arbuscular mycorrhizal fungi grow 10–20 times larger than nonmycorrhizal plants growing in sterile soil.

Box
ARBUSCULAR MYCORRHIZAL FUNGI AND CASSAVA.
OPTIMIZATION OF SOIL MICROBIAL COMMUNITIES COULD ALLOW FARMERS TO APPLY LESS CHEMICAL FERTILIZER, THUS SAVING MONEY AND REDUCING THE AMOUNT OF EXCESS NUTRIENTS THAT LEACH OUT OF FIELDS INTO WATER SYSTEMS.
Other nutrients, like sulfur, potassium and iron can also be transformed into usable forms or transported to plants by both bacteria and fungi. Optimization of soil microbial communities could allow farmers to apply less chemical fertilizer, thus saving money and reducing the amount of excess nutrients that leach out of fields into water systems.
PATHOGEN AND PREDATOR RESISTANCE

Plants are subject to many potential biological enemies, including bacterial, fungal and viral pathogens that cause disease as well as parasites, insects, birds, and grazing animals that feed on plants. Partnerships with microbes can help plants resist these threats.
In the simplest case, microbial partners may simply occupy niches that otherwise might be vulnerable to pathogens. When bacteria form a biofilm around the roots of a plant, microbial pathogens and soil–dwelling parasites cannot gain access. The beneficial microbes in the rhizosphere may be doing more than just getting in the way; they may also be producing any one of a number of chemicals that act directly against pathogens. Many of the antibiotics humans use to treat infections are derived from bacteria or fungi that produce them to kill or inhibit competing microbes. Other microbially-produced chemicals may serve to mask the presence of the plant from would be parasites or predators, attract beneficial organisms, or stimulate the plants' immune system. Bacteriophage — viruses that infect bacteria — may kill pathogenic bacteria directly. There is even evidence that microbes can generate electrical fields that can attract or deter other microbes and soil invertebrates like nematodes.
Above ground as below, microbes can serve the plant simply by occupying space that otherwise might be an entry point for pathogens. Surface and endophytic microbes also make a variety of potentially helpful compounds including toxins that deter grazers, volatile compounds that alert neighboring plants to the presence of a threat, and small molecules that trigger protective responses like the closing of stomata.
There is crosstalk between the “conversations” going on between plants and the microbial communities above and below ground; events in the soil can trigger responses in leaves and vice versa. For example, when tomato plants are attacked by the early blight fungus, their activated immune system produces stress signals that are perceived by fungi that live around the roots. Through their hyphae, the beneficial root fungi then transmit the stress signal to neighboring plants warning them to up-regulate their stress responses.
Microbes produce a wide array of compounds that inhibit or kill competing microbes. If a bacterium, virus or fungus can deter another microbe that is harmful to a plant, it may well be in the plant's interests to provide the helpful microbe with shelter and nourishment. It is likely that many plant-microbe partnerships have evolved on this basis: the plant supplies carbohydrates to certain microbes in return for the deterrence of pathogens or predators or other benefits. It has been estimated that up to 30% of a plant's primary production (that is, the amount of carbon the plant turns into organic matter through photosynthesis) actually leaves the plant as exudate into the soil; the microbes must be making a fairly substantial contribution to earn such a high investment of the plant's resources.

Box
COST-BENEFIT ANALYSES IN PLANT-MICROBE INTERACTIONS.
RESISTING ENVIRONMENTAL STRESS
If, as just noted, microbes can earn a living protecting plants from predators, it stands to reason that microbes might also benefit from helping the plant survive times of environmental stress. Indeed, microbes have been shown to be important partners in mitigating the effects of virtually every known environmental stress that can affect plants.

- ▪ Drought — Plants need water to maintain cell structure and drive biochemical reactions like photosynthesis. Under drought conditions, plants limit water losses by closing stomata (openings on the undersides of their leaves), but this prevents proper temperature regulation and capture of CO2 for photosynthesis. Epiphytic and endophytic fungi help to relieve this stress by stimulating plant root growth, thus increasing the efficiency of water uptake from the soil. Acute plant viruses that under normal conditions cause disease have also been shown to improve drought tolerance in infected plants.
Figure
DROUGHT
- ▪ Flooding — Although plants need water to survive, too much of a good thing can be detrimental. Indeed, flooding drives plant roots towards hypoxia or oxygen limitation. In response to several stresses, including flooding, plants produce ethylene, which activates defense and protective mechanisms but can also shut down root and shoot growth. Many species of bacteria express the enzyme ACC deaminase, which degrades ACC, the precursor to ethylene. By blocking ethylene production, these microbes allow continued root development and increase plant growth in the presence of flooding or drought.
Figure
FLOOD
- ▪ Salinity — Excessive salts in the soil mimic drought conditions for the plant, as they pull water out of plant tissues. Salinity inhibits seed germination, seedling growth, flowering, and fruit set among other effects. Fungal endophytes isolated from dune grass on the Pacific coast confer salt tolerance, as do some rhizobacteria, whose exopolysaccharide secretions potentially bind sodium and other cations, preventing their uptake by the plant.
- ▪ Heavy metal contamination — Heavy metal contamination in soil and ground water can interfere with ionic homeostasis in plants, and additionally cause cellular damage through oxidative stress. Bacteria can both decrease toxicity of these metals and promote plant growth by sequestration of the metals themselves, rendering them biologically inactive, or also inhibiting the overall plant ethylene stress response through ACC deaminase activity.
- ▪ Organic pollutant contamination — The range of biosynthesis and biodegradation pathways found in bacteria is enormous. No matter what the pollutant, there are almost certainly bacteria somewhere that can modify or degrade it. When soil is contaminated, a partnership between bacteria that can degrade or detoxify a compound that is inhibiting plant growth allows those bacteria to reproduce more rapidly. Moreover, their association with plant roots increases their access to pollutants due to pollutant movement to roots via the transpiration stream, and also helps transport these bacteria through the soil.
Figure
POLLUTION
- ▪ High temperatures — All enzymes have temperature ranges in which they function best. Temperature fluctuations above these thresholds can deactivate or destroy these proteins, leading to decreased growth. Even a 1°C increase in nighttime temperature can decrease rice productivity by 10%. However, potatoes grown in the presence of rhizobacteria exhibit increased root and shoot growth as well as increased tuberization under heat stress.
- ▪ Low temperatures — Cold temperatures result in slowed growth, and can even damage cell membranes. Although the mechanisms underlying microbial protection are unclear, grapevines inoculated with Burkholderia species of bacteria have increased photosynthesis rates and decreased amounts of electrolyte leakage (indicative of membrane damage) relative to control plants in cold temperatures.
Figure
FROST

Box
THE UNTAPPED POTENTIAL OF MICROBIAL BIODIVERSITY.
NORMAL GROWTH AND DEVELOPMENT
Scientists are still unraveling the many ways that plants can make use of microbial capabilities to make nutrients more available or deter pathogens. Perhaps even more surprising, discoveries are emerging from the realization that microbes play critical roles in plant development, physiology and metabolism. These roles often are carried out through microbial synthesis of biochemicals that mimic, amplify, inhibit, or modify the activities of plant hormones. For example, bacteria and fungi produce a number of phytohormones that increase root hair production, which increases the absorption capacity of the roots. Some seeds require bacteria to germinate. Other microbial compounds enhance root respiration or affect transpiration by modifying the activity of stomata. The actions of these compounds are not entirely dependent on physical contact with the plant; fungi, in particular, produce many volatile compounds that can act at a distance both on neighboring plants and on other ecosystem inhabitants like insects.
FLAVOR
Microbes can even affect the flavor of food plants. In strawberries, the methylotrophic bacterium Methylobacterium extorquens enhances the production by the plant of chemicals called furanones that are responsible for the characteristic flavor of strawberries. One of these furanones — 2,5-dimethyl-4-hydroxy-2H-furanone (DMHF) — also stimulates plant defenses, increasing plant production of various antimicrobial compounds to deter harmful microbes.
THERE ARE MULTIPLE, LIVELY CHEMICAL CONVERSATIONS GOING ON UNDER OUR FEET…
As all of these examples demonstrate, there is much more going on in a plant's world than meets the eye. A complex but largely invisible ecosystem surrounds each plant that includes bacteria, fungi, viruses, and soil invertebrates with multiple, interwoven networks of predation, pathogenesis, cooperation and interdependency. There are multiple, lively chemical conversations going on under our feet, conversations that include some overlapping vocabulary and unambiguous signals, but also numerous efforts to encrypt, deceive, or eavesdrop. The conversations connect plant to plant, plant to microbe, plant to nematode, microbe to microbe, nematode to nematode and every other conceivable combination — with viruses potentially participating in multiple ways. The inhabitants of these ecosystems can listen in on some of each other's conversations, interfere with others, impersonate one another, and amplify or dampen signals; a single chemical can mean different things to different organisms or at different times. In short, there is a great deal of communication going on and we are just beginning to realize how important it is. A goal for the future is to be able to listen in ourselves.

HOW DO PLANTS AND MICROBES INTERACT?

The interactions between plants and microbes are not exclusively chemical, although small molecules are an important “common language.” Plants produce flavonoids that attract and repel various microbes, while microbes produce Nod factors that induce root differentiation. Both plants and microbes produce volatile organic compounds that can act locally or at a distance.
PLANTS CAN PRODUCE QUORUM SENSING MIMICS AS WELL AS QUORUM INHIBITORS IN AN EFFORT TO SKEW BACTERIAL BEHAVIOR IN A DIRECTION BENEFICIAL TO THE PLANT.
Bacteria produce a number of small molecules that serve as quorum sensors — that is signals that bacteria use to determine the density of the local bacterial community and how many of their own species are present. Bacteria integrate these signals to cue group behaviors like biofilm formation or toxin secretion. Plants can produce quorum sensing mimics as well as quorum inhibitors in an effort to skew bacterial behavior in a direction beneficial to the plant. A South American flowering plant called Gunnera, in a process analogous to the establishment of root nodules in legumes, produces a small molecule that induces nitrogen-fixing bacteria to differentiate into a highly mobile filamentous form. This form migrates into a cavity within the plant's stem where it establishes a colony that provides the plant with fixed nitrogen in exchange for sugars.

Box
BIOTECHNOLOGY POWERHOUSES: ANOTHER REASON TO STUDY THE MICROBES THAT ASSOCIATE WITH PLANTS….
Another way that the various microbial organisms living around plants can interact both with plants and with one another is through direct transfer of genetic material. Bacteria exchange plasmids with each other via the process of conjugation, and viruses shuttle genes from one organism to another on a regular basis. Direct transfer of genetic material from Agrobacterium to plants also occurs. The genomes of organisms reveal numerous examples of gene exchange over evolutionary history, but the frequency, timing and triggering conditions that govern these exchanges are not known. Bacteria of the genus Agrobacterium can participate in the process of conjugation with plant cells; the plasmid they transfer integrates into the plant genome where it expresses genes, including the plant hormones auxin and cytokinin that induce the plant to form a tumorous growth called a gall. This capability has been exploited in biotechnology to transfer desirable genes into plants. Viruses also can be engineered to transfer desirable genes into plants and bacteria.
Another form of interaction is the transfer of proteins between organisms. Bacteria and fungi both produce proteins called effectors that they inject into plant cells. Most of the effectors that have been characterized so far are produced by parasites or pathogens and act to suppress the plant's innate immune system. Determining whether there are beneficial effector-like interactions is an interesting topic for future research.
As touched on above in the discussion of chemical and genetic transfer, a great deal of interaction between plants and microbes uses the language of plant hormones. Microbes produce a number of plant hormones, and they also produce enzymes that alter plant hormone levels. In return, plants produce compounds to block or modify the bacterially produced plant hormones.
Finally, some of the interactions between and among plants and microbes are purely “mechanical” or physical; some beneficial microbes simply occupy space that otherwise might be vulnerable to pathogens. The morphology of plant leaves, flowers and roots can play a part in which microbes can survive. For example, microbial nitrogen fixation is inhibited by the presence of oxygen, so the provision by the plant of a protected physical space is crucial to that particular plant-microbial partnership.

CURRENT APPLICATIONS
Using microbes to boost plant production or protect plants from pests is not a new idea. Indeed, traditional agricultural practices like crop rotation or co-cultivation of legumes with non-leguminous plants have been in use for millennia. There is even a small, but significant market for biological crop treatments. Biopesticides, a category that includes biological agents used as insecticides, herbicides, pesticides, and fungicides have been estimated to constitute a $2.1 billion per year industry — about 5% of the $44 billion per year chemical pesticide industry. A little over half of that market is made up of live microbes; other types of biopesticides include non-microbial agents like insects and biologically-derived compounds like pheromones. The global market for chemical fertilizers is on the order of $150 billion per year; microbial approaches could potentially substitute for or augment this market.
BACTERIA, FUNGI, AND VIRUSES HAVE ALL BEEN ADAPTED AND MARKETED FOR AGRICULTURAL PURPOSES INCLUDING PROTECTION AGAINST PATHOGENS AND PESTS AND FOR GENERAL PLANT HEALTH AND PRODUCTIVITY.
Bacteria, fungi, and viruses have all been adapted and marketed for agricultural purposes including protection against pathogens and pests and for general plant health and productivity. Categorization is tricky. If a microbe is helping the plant by discouraging root growth in competing species, does that make it an herbicide? If a microbe spurs root growth, and thereby boosts plant productivity, does that make it a fertilizer? In some cases, the mechanisms by which a microbe is helping the plant may be unknown, or the microbe may have more than one effect. Sometimes, there are regulatory and marketing reasons to emphasize one aspect of microbial activity over another. For example, products marketed as pesticides face a lengthy approval process that can be avoided by emphasizing a microbial treatment's ability to boost productivity rather than its ability to deter pests.
There are lessons to be learned from early successes in marketing microbially-based products for agriculture. The following characteristics were critical to success:
- ▪ Well-characterized microbes and clear understanding of their mechanism of action.
- ▪ A high level of specificity in the plant-microbe interaction — for example, the rhizobium-legume symbiosis.
- ▪ Biological simplicity — for example, Bt toxin is coded for by a single gene that can be easily transferred into the plant genome.
- ▪ A defined window of susceptibility — Agrobacterium can successfully transfer genes into plant cells when applied in the first 24 hours after the plant is wounded.
- ▪ Strategies to overcome challenges in scaling up for commercial production, obtaining regulatory approval, and ensuring adequate shelf-life.
- ▪ Identification of conditions for maximal efficacy and product consistency.
- ▪ Functional collaborations combining expertise in basic science, development, testing, and marketing.
- ▪ Outreach to end-users including technical support and education, particularly with respect to disease prevention rather than treatment following disease outbreaks.
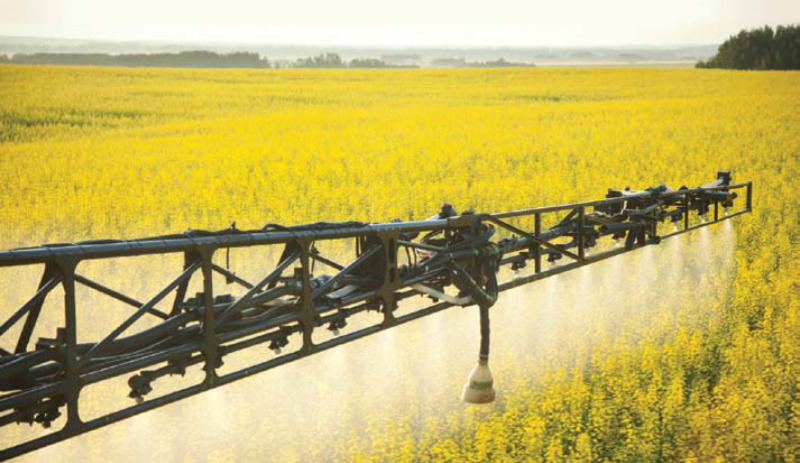
Not all microbe-based treatments are implemented in the same way, and the choice of implementation model has implications for how long and costly the development process will be. The most time-consuming and costliest approach is full registration as a pesticide, following the same regulatory pathway as chemical pesticides. If an organism is being introduced solely to control a pest or pathogen, this is the appropriate route, but it costs up to $10 million and can take four to six years to accomplish. For microbial treatments aimed at plant strengthening or biofertilization, registration is not required, costs drop nearly ten-fold, and time to market can be reduced to one or two years. Another model is that of local production, in which the microbial agents or their extracts are produced and applied locally rather than purchased from an external supplier. This model can be a win-win situation for communities; local fermentation or cultivation facilities provide jobs while farmers gain access to treatments tailored to their specific crops and conditions. Direct government support of such production facilities has been implemented in a few countries, including Venezuela and Cuba.
PUTTING MICROBES TO WORK
The potential applications of beneficial microbes in agriculture seem boundless, but progress will require advances in basic understanding of plant-microbe interactions coupled to practical attention to the process of moving discoveries from the lab to the field, as well as technical, regulatory, marketing, and end-user education issues. An integrated approach to addressing these challenges — in other words, an approach that acknowledges the multi-faceted nature of the challenges — is most likely to succeed.
WE ARE NOT USED TO CONSIDERING THE MEMBERS OF ECOSYSTEMS THAT FUNCTION AT THE MICROSCOPIC SCALE. THEIR ACTIVITIES, THOUGH INVISIBLE, ARE ESSENTIAL TO THE OVERALL FUNCTIONING OF THE ECOSYSTEM.
The scientific challenges facing this field require a change from the past when most studies of beneficial plant-microbe interactions focused on one plant-microbe partnership at a time. Such reductionist approaches are critically important and many more of them will be needed to elucidate the mechanisms of interaction between particular plants and individual microbes. However, progress will also require getting into the mindset of understanding every organism as a member of a series of interacting ecosystems. We are familiar with large-scale ecosystems, for example, a grassland where herbivores, predators, scavengers, plants, pollinators, and decomposers constitute a complex food web. Ecological studies in such macro-ecosystems have demonstrated how removal of one species can affect the other relationships in complex ways, as can the introduction of a non-native species. We are not used to considering the members of ecosystems that function at the microscopic scale. Their activities, though invisible, are essential to the overall functioning of the ecosystem.
SCIENTIFIC CHALLENGES
IDENTIFYING THE PLAYERS
The staggering diversity of microbes found in the soil makes identifying the players a daunting task. Identifying each microbe in every environment is clearly an unattainable goal, especially since the systems are dynamic. Nevertheless, for any given plant system, a systematic survey of the members of the community is becoming possible with metagenomic approaches. A comprehensive catalog of the microbial community associated with any individual plant species, including a major crop species, has not been performed; therefore, “census-taking” activities are currently warranted to establish broad patterns. Metagenomic techniques are well-developed for bacteria; techniques for assessing the viral and fungal inhabitants of plant-microbe communities are less well-established. Another layer of complexity is added when the invertebrate members of these communities — the insects and nematodes that interact with the plants and microbes in numerous ways — are taken into account.
UNDERSTANDING THE SIGNIFICANCE OF DIVERSITY
EFFORTS TO “KNOW WHO IS THERE” MUST BE ACCOMPANIED BY AN EFFORT TO UNDERSTAND WHAT FRACTION OF THAT DIVERSITY IS TRULY REQUIRED FOR OPTIMAL PLANT HEALTH IN ANY GIVEN ENVIRONMENT.
Technical advances are making it easier to take a census of a soil community. But efforts to “know who is there” must be accompanied by an effort to understand what fraction of that diversity is truly required for optimal plant health in any given environment. The vast diversity of microbes in the soil probably results from the ability of many microbes to survive at very low population levels, ready to multiply when conditions are favorable. The consequence for plants is that there are a host of microbes available to provide needed capabilities as environmental conditions change. To researchers seeking to understand the key drivers in the system, the diversity is daunting. There is undoubtedly a great deal of functional redundancy among different soil microbes. If this were not the case, it would be dramatically more difficult to grow plants outside of their native habitat.
On the other hand, research on arbuscular mycorrhizal fungi has shown that while cassava is never found without AMF in the wild, different species of AMF have dramatically different effects on cultivated cassava, ranging from no effect to a 20% increase in yield. Thus, it would appear that while a general function can be supplied by a number of different species, for example, phosphate acquisition by AMF or nitrogen fixation by bacteria, all members of a functional group are not necessarily equal and some can have impacts above and beyond those of their genetic neighbors. Determining which specific genotypes are optimal and why will be a significant scientific challenge.
Much of the microbial diversity in the soil is probably not active at any given time, thus the impact of the lack of certain species or functional groups might be minimal under most circumstances. Entirely unrelated microbes can fulfill the same ecological roles at different times or in different places and, conversely, one microbe may fill different roles depending on the circumstances. The potential contribution of certain microbes may be masked when conditions are favorable, or inputs like water and nutrients are being provided by the farmer. Answering the question of whether there is a common “minimal microbiome” for plants — that is, a small set of microbes that can provide all essential services — will have significant implications for optimizing agricultural conditions.
CHARACTERIZING FUNCTIONAL CAPACITY
Metagenomics and transcriptomics techniques, which derive information from gene sequences, can provide information about “who is there” and, to some degree, “what they are doing,” but in any environment, there is an enormous knowledge gap between acquiring the gene sequences and knowing what the gene products do, which organisms they come from, and what regulates their activity. This challenge is magnified in the exceptionally diverse soil environment. Determining what capabilities are present in any given soil and what aspects of the community diversity are essential to function is a major challenge. Getting a handle on functional diversity will be very important to accessing the capacity of microbes to enhance plant productivity.
UNDERSTANDING INTERACTION DYNAMICS
We know of a few individual effects that plants and microbes have on each other, but we are very far from having a map of all interactions or being able to predict how the various interactions combine to produce a particular phenotype. As noted earlier, these interactions are of various kinds — chemical, genetic, physical — and are highly dynamic. Manageable approaches to ‘listening in’ on these many conversations might include new visualization techniques and new tools for connecting ‘chemotype’ (the collection of chemical compounds flowing among community members) to ‘phenotype.’

UNDERSTANDING RESILIENCE
If the ultimate goal is the development of interventions that improve plant productivity and resistance to disease and pests, it will be necessary to figure out at which points these complex systems are susceptible to manipulation. Treatments that work in the simplified conditions of the laboratory frequently fail when they are tested in the more complex conditions of the field. This may be due in part to competition from the established microbial communities, the absence of necessary partners, or inauspicious environmental conditions or timing. Being able to manipulate plant-microbe communities predictably will require advances in our understanding of how communities evolve over time and which characteristics are most amenable to reproducible manipulation.
PUBLIC ACCEPTANCE OF MICROBIAL SUPPLEMENTS WILL REQUIRE THAT THE ENVIRONMENTAL IMPACT OF ADDING MICROBES BE THOROUGHLY EVALUATED.
Understanding the resilience of microbial communities will also be necessary to assess the risks and benefits of introducing novel microbes in agricultural settings. Public acceptance of microbial supplements will require that the environmental impact of adding microbes be thoroughly evaluated and that the mechanisms for enacting beneficial community changes be understood. For example, do introduced microbes alter the diversity of the local microbial communities? Do they cause shifts in native microbial community structure? Are such changes beneficial or detrimental to plant health or sustained productivity? Could introduced microbes spread beyond the target crop, and would that be cause for concern? Advances in fundamental understanding of community dynamics will be needed to answer these important questions.
DISCOVERING CROP PLANTS' EVOLUTIONARY PARTNERS

EVOLUTIONARILY OPTIMIZED MICROBIAL COMMUNITIES ALSO MIGHT ALLOW CROP PLANTS TO GROW IN POORER SOIL OR UNDER THE LESS PREDICTABLE CLIMACTIC CONDITIONS THAT WILL ACCOMPANY CONTINUED CLIMATE CHANGE.
The microbial context in which the ancestors of current crop plants evolved surely differed from that in which their descendants are currently grown. Understanding the partners with which each plant evolved is a highly promising target of intervention to improve crop productivity. It seems clear that plants are able to recruit local microbes for many functions, but it is possible that providing plants with microbial partners more closely related to those with which the plant evolved could allow a dramatic reduction in the artificial inputs — including chemical fertilizers, pesticides, and herbicides — that humans have employed to help plants grow in new environments. Evolutionarily optimized microbial communities also might allow crop plants to grow in poorer soil or under the less predictable climactic conditions that will accompany continued climate change.
BREEDING FOR SUCCESSFUL PLANT-MICROBE INTERACTIONS
For millennia, plant breeders have taken natural genetic variation in plants and used it to breed new varieties, and these techniques have grown increasingly more sophisticated. However, breeding to optimize interactions with known beneficial microbes has not been attempted, but is as likely to succeed as breeding for any other desirable trait. Similarly, or perhaps even better, it should be possible to “breed” beneficial microbes so that they interact more successfully with a given crop plant. To date, microbes that have been discovered to be beneficial in one context are then marketed as general crop boosters. Often they fail to provide consistent benefits in other soils. However, there is no reason not to take advantage of genetic variation in populations of these microbes and use that to breed better microbes for specific applications. This approach could be even more rapid than plant breeding given how quickly microbes reproduce. In short, plant breeding can now explore a new trait for optimization and microbial breeding programs for crop improvement should be considered.
VITALLY NEEDED NEW TOOLS AND APPROACHES
MORE, FASTER, AND CHEAPER ‘OMICS
High-throughput nucleic acid sequencing technologies like metagenomics and metatranscriptomics are revolutionizing our ability to determine which organisms are present and which genes are being expressed. The faster, cheaper, and more accessible these techniques become, the easier it will be to discern patterns in the microbiomes of various plants in multiple environments.
But these nucleic acid based techniques are only the beginning. It is a long road from sequence information to understanding community dynamics, metabolism, and responses to perturbation. Proteins and small molecules may provide a much more direct read-out of community activity than gene sequences, but the technologies for generating proteomes and metabolomes lag far behind those for nucleic acid sequencing. The ability to query other molecules in a high-throughput manner would be transformative.
BETTER ANNOTATION
As genomic and transcriptomic datasets accumulate, identifying the functions of the enormous number of currently uncharacterized genes is becoming increasingly important. The combination of efforts to characterize gene function with models that predict key genes for target ecological processes will be particularly powerful. High throughput methods for gene annotation, discovery and functional prediction are urgently needed.
As fungal and viral components of plant-microbe communities are increasingly included in microbial community analyses based on gene sequence, the problem of unannotated genes and genes of unknown function is exploding. However, chances are good that study of these relatively neglected organisms will reveal new metabolic and synthetic pathways, and novel strategies to deal with universal biological imperatives like pathogen and stress resistance. Learning more about gene function in these organisms will be rewarding in itself, and no doubt crucial to understanding the overall dynamics of plant-microbe ecosystems.
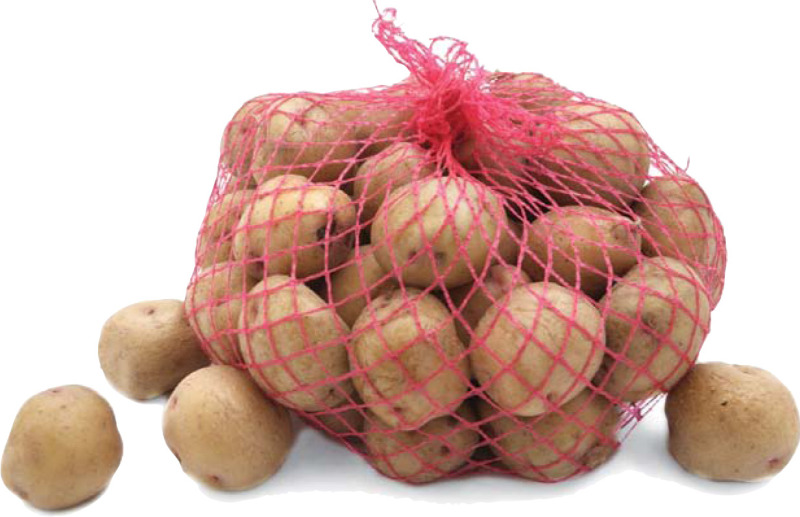
MORE MODELS AT ALL SCALES
Models are available for many individual microbes, as well as for some plants (Arabidopsis, maize). Fundamental research is needed on how to characterize and quantify different kinds of interactions, infer system behavior from those interactions, and predict how individual organisms and the overall system will react to perturbations. Models could advance study of any number of relationships, for example, the role of individual microbes in providing nutrients given environmental conditions, microbe-microbe interactions, microbe-plant interactions (both symbioses and endosymbioses), and multi-species systems. Understanding complex plant-microbe interactions at a systems level will require a collection of linked models spanning scales from individual organisms to pair-wise interactions to network analysis. The ultimate goal is integration of the models at the various levels to provide a realistic picture of system behavior.
Models are most powerful when they are developed in concert with experimental approaches. Iteration between observed results and theory — that is, combining ‘top-down’ and ‘bottom-up’ approaches — advances understanding more quickly. A true systems biology of plant-microbe interactions will require a robust computational foundation.
TECHNIQUES FOR STUDYING THE RHIZOSPHERE
ROOTS ARE DOING A LOT MORE THAN HOLDING PLANTS IN PLACE.
Roots are doing a lot more than holding plants in place. The myriad interactions taking place among plant roots, their exudates, and the thousands of species of microbes in the soil are fundamental to plant health and productivity. They remain, however, to a large degree, a black box. Methods for studying these interactions on a molecular level and in real time and space, are essential in order to determine the optimal community for plant productivity and successfully optimize the soil microbiome in the field.
AGREED METRICS OF PRODUCTIVITY AND YIELD
An issue that is more complicated than it appears is the need to develop agreed-upon measures of ideal productivity and yields. Only with good quantitative metrics will it be possible to demonstrate the value of microbially-based interventions.
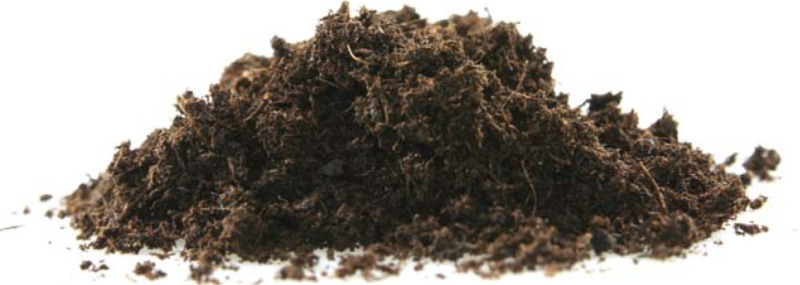
GETTING DISCOVERIES INTO THE HANDS OF FARMERS
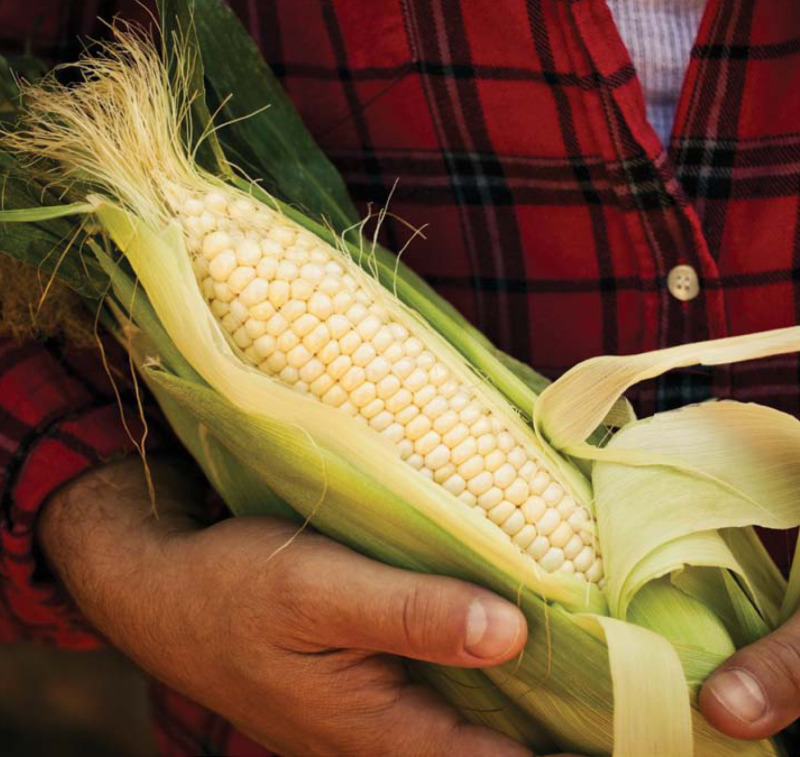
In all areas of science, there are challenges in translating advances in basic knowledge into new products and practical applications. The area of plant-microbe interactions is no different, and, in fact, past experience has left a legacy of skepticism about whether discoveries in this area can be applied in the “real world” of farmers' fields.
A burst of interest and research several decades ago in the topic of biocontrol — using microbes to protect plants from disease — led to some successful products, but many microbe-based treatments that showed promising results in the controlled conditions of the laboratory or greenhouse were unsuccessful when applied in the field. In light of the emerging evidence that plant-microbe interactions are complex and highly interdependent, it is likely that earlier failures resulted from an inability to predict the conditions that would allow an introduced microbe to become established and provide the desired service. It is now clear that introduction of a single microbe may be ineffective if a critical partner is not present, or if a competitor is occupying the target niche. Overcoming this hurdle will not be trivial, but awareness of the complexity and dynamism of these ecosystems is an important first step. Because so many advances have been made in the last 20 years and so many new technologies are now available, it is time to take a fresh look at this field.
Microbial ecology is undergoing a renaissance and new tools are opening up many environments to study. From the ocean to the human gut, researchers are learning more and more about how complex microbial communities form and change over time. The soil environment is often characterized as the most complicated of all, and perhaps the most difficult to study. But it is likely that many underlying principles are shared among these microbial assemblages and soil microbial ecology will benefit from advances in other environments.
THE MOMENTUM OF THE SCIENCE, COMBINED WITH THE UNDENIABLE URGENCY OF THE PROBLEM, SUGGEST THAT THE TIME IS RIPE FOR INVESTMENT IN PLANT-MICROBE INTERACTIONS WITH THE GOAL OF IMPROVING AGRICULTURAL PRODUCTIVITY.
The momentum of the science, combined with the undeniable urgency of the problem, suggest that the time is ripe for investment in plant-microbe interactions with the goal of improving agricultural productivity. If this is the case, how best should that investment be structured? The participants in this colloquium agreed that a three-step approach, modeled loosely on the clinical trial approach used for biomedical treatments, would be most likely to bear fruit in a timely manner.
RECOMMENDATION 1: INVEST IN CURIOSITY-DRIVEN, FUNDAMENTAL RESEARCH
There is a central and inevitable conundrum at the heart of all calls to enhance investment in a particular area of basic research. Experience tells us that while virtually all innovative products rely on advances made in basic research, it is impossible to predict which fundamental research projects will yield results most quickly. A formula that has been enormously successful for the last six decades has been public support of investment in investigator-initiated, curiosity driven projects, with projects chosen based on peer-review.
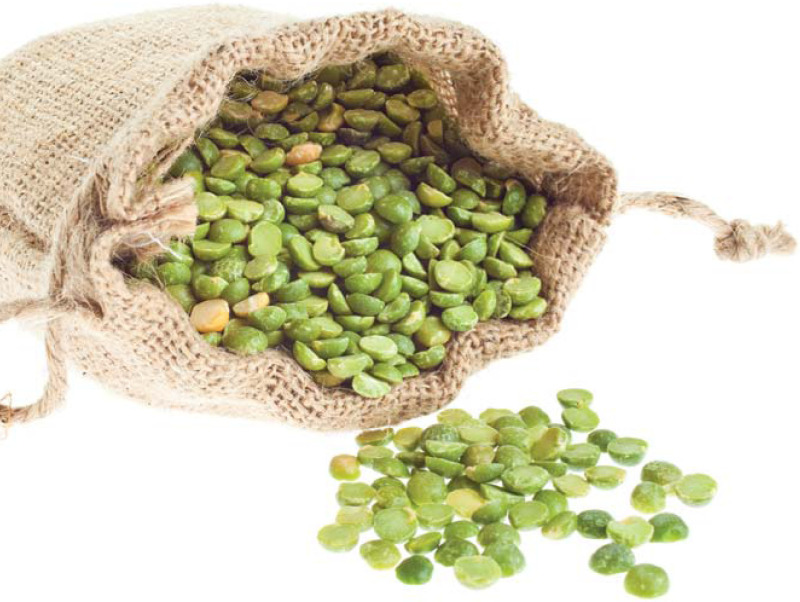
RECOMMENDATION 2: TAKE ON ONE OR MORE GRAND CHALLENGES
While acknowledging that important discoveries are likely to come from the small science approach, the field of beneficial plant-microbe interactions is exceptionally broad, and research funding is not unlimited. Setting a major challenge toward which individual projects could aim can be inspiring, both for scientists and the general public, and can be structured to include investment in infrastructural and technological needs that benefit the wider scientific community as well as those working on the grand challenge itself. A portfolio of funding opportunities ranging from curiosity-driven, single-investigator projects, to targeted tool development, to the establishment of teams or centers, can all be used to meet a challenge. Grand challenges are most successful if they are more ambitious than could be achieved by conventional funding approaches, but still realistic. A measurable endpoint that represents successful completion of the challenge is highly desirable. In the area of plant-microbe interactions, many such goals are possible, within which the scope for curiosity-driven research would be great. Some significant and achievable challenges identified during the colloquium were:
- ▪ 20:20 in 20 — increase yields by 20% while reducing fertilizer and pesticide use by 20% in 20 years: The most practically focused of the challenges would call for research that contributes to increasing crop yields while simultaneously reducing demand for external inputs. Colloquium participants agreed that the goal of increasing productivity by 20% while similarly reducing chemical inputs, is ambitious, but attainable, with dramatic benefits for consumers, farmers, and the environment.
- ▪ Fully characterize the microbiome of a single important crop plant: Such a project would examine the microbial partners of a particular plant across various parameters — how do the microbial partners vary in different geographic areas, at different stages of development, from strain to strain, under different treatment and cultivation regimes? What were the microbial partners of the wild ancestors of the crop plants? How do the microbial communities associated with the plant react to changes in temperature, moisture and atmospheric CO2 levels and influence the responses of the plants to these changes? What are the biological properties of the microbial partners and is there a minimal or core microbiome? Can the introduction of specific microbes have a predictable influence on the biology or performance of the host plant?
- ▪ Characterize the response of a variety of plant-microbe communities to a particular stress: Including both cultivated and wild plant-microbe systems, such a project would focus on multiple responses to a single stressor like drought, salinity, or nutrient limitation.
RECOMMENDATION 3: ESTABLISH A FORMAL PROCESS FOR MOVING DISCOVERIES FROM THE LAB TO THE FIELD
- ▪ Encourage researchers to identify potential applications of basic discoveries: Investment in curiosity-driven research will certainly result in discoveries with practical applications. Help should be available for researchers who wish to explore the possibility of moving their discoveries into the application pipeline. The goal would be to elicit a wide diversity of approaches, with advancement to the next stage based on peer review.
- ▪ Support a large number of pilot projects aimed at evaluating treatments based on basic discoveries: Projects at this stage would be evaluated both for efficacy and safety, with agreed standards and protocols for measuring outcomes and assessing risks. Not all treatments would involve the introduction of novel viable organisms; in some cases, it may be possible to change external conditions in a way that favors the establishment or resilience of an optimal microbe-plant community. Agreed standards for assessing the risk of introduced organisms will be essential for subsequent regulatory and public approval. Involving a broad base of stakeholders at this stage, including potential users, extension agents, regulatory agencies, consumer groups and others, is likely to be helpful. Building awareness of how microbes and plants interact and discussing of the potential risks and benefits of strategies for fostering beneficial interactions will contribute to more successful adoption when new applications reach the commercial stage.
- ▪ Comprehensive field trials of the most successful pilot projects: As outlined above, successful efforts to make use of microbes in agriculture resulted from knowledge of particular microbes, microbial functions or plant-microbe interactions. However, additional factors in the commercialization process play a large role in the successful translation of a particular discovery.. These factors include technical issues like scaling up production and maintaining viability through the supply chain, business concerns like navigating intellectual property allocation and regulatory approval, and societal concerns like farmer receptivity and consumer acceptance. Even a stunning scientific advance is only the first step toward the transfer of knowledge to enhanced agricultural productivity and sustainability. Success stories indicate that including all of the stakeholders throughout the process is valuable.
THE PROCESS OF DEVELOPING SUCH PROTOCOLS WOULD IN ITSELF BE AN OPPORTUNITY FOR THE VARIOUS STAKEHOLDERS — SCIENTISTS, FUNDING AGENCIES, REGULATORY AGENCIES, PRIVATE INDUSTRY, EXTENSION PROGRAMS, AND CONSUMER GROUPS — TO BEGIN TO WORK WITH EACH OTHER PRODUCTIVELY.
Because these non-scientific factors weigh so heavily in the ultimate success of translating discoveries to application, it would be helpful to develop systemic, standardized, phased protocols for translation from the laboratory to the field. The process of developing such protocols would in itself be an opportunity for the various stakeholders — scientists, funding agencies, regulatory agencies, private industry, extension programs, and consumer groups — to begin to work with each other productively.
CONCLUSION
Achieving food security for a still-expanding global population is a large and complex challenge. Increasing agricultural productivity without increasing pressure on natural environments or consuming more fossil fuels is a goal that will require progress across many fronts. Sometimes, however, a great need and a new opportunity happen to coincide and the colloquium discussions summarized in this report demonstrate that now is such a time. All plants, in all environments, depend on microbes, and therefore, potentially all crops, no matter where they are grown, could benefit from optimization of their microbial partners. The time is right to enlist the capabilities of the microbial world to help solve this pressing human problem.
This work is licensed under a Creative Commons Attribution-NonCommercial-NoDerivatives 4.0 International License.