This work is licensed under a Creative Commons Attribution-NonCommercial-NoDerivatives 4.0 International License. To view a copy of this license, visit https://creativecommons.org/licenses/by-nc-nd/4.0/
NCBI Bookshelf. A service of the National Library of Medicine, National Institutes of Health.
Gilmore MS, Clewell DB, Ike Y, et al., editors. Enterococci: From Commensals to Leading Causes of Drug Resistant Infection [Internet]. Boston: Massachusetts Eye and Ear Infirmary; 2014-.
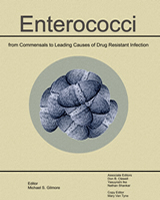
Enterococci: From Commensals to Leading Causes of Drug Resistant Infection [Internet].
Show detailsEnterococcal Bacteriophages
A brief overview of bacteriophages: Bacteriophages (phages) are viruses that infect bacteria. Similar to the viruses of plants and animals, phages are inert and are unable to propagate themselves in the absence of a host. Phages depend on host metabolism to provide the organic material and machinery necessary for their replication and for the subsequent packaging of the viral genetic material during phage particle biosynthesis. Phages are associated with nearly all known bacterial taxa and, as a result, are found in diverse environments that range from soil to oceans and even in deserts (Prestel, Salamitou, & DuBow, 2008; Prigent, Leroy, Confalonieri, Dutertre, & DuBow, 2005; Srinivasiah, Bhavsar, Thapar, Liles, Schoenfeld, & Wommack, 2008; Wommack & Colwell, 2000). Phages are found either directly associated with their bacterial hosts or in large numbers as free virions in the environment. Since there is a vast distribution of phages across the globe, it is possible to theorize that phages constitute the most abundant biological entities on earth. Their numbers have been estimated to reach as high as 1031 particles with the potential for 1025 phage infections occurring every second (Pedulla, et al., 2003; Wommack & Colwell, 2000). As many more phage genome sequences have become available in recent years, it is obvious that phages are extremely incongruent at the genomic level. This diversity in genetic makeup is proposed to result from the fastidious replication of phage particles during the infection of highly permissive hosts. During these infections, phages are able to exchange DNA within host genomes through recombination, and continually generate diversity as a result (Hendrix, Smith, Burns, Ford, & Hatfull, 1999).
The vast majority of phages belong to the order of Caudovirales, which are tailed phages that have dsDNA and an isometric capsid. Caudovirales is comprised of three phylogenetically-related families that are discriminated by tail morphology: Myoviridae (long contractile tails), Siphoviridae (long non-contractile tails), and Podoviridae (short tails) (Ackermann, 2007; Krupovic, Prangishvili, Hendrix, & Bamford, 2011). The most well-studied tailed phages are the coliphages ʎ (Siphoviridae), T4 (Myoviridae), and T7 (Podoviridae) which infect Escherichia coli and which have served as workhorses for elucidating the mechanisms of modern molecular genetics and biochemistry (Johnson, Poteete, Lauer, Sauer, Ackers, & Ptashne, 1981; Miller, Kutter, Mosiq, Arisaka, Kunisawa, & Rüger, 2003; Ptashne, et al., 1980; Tabor & Richardson, 1985). Far less abundant are the non-tailed phages, which encompass numerous families with great morphological distinction; these include phages that are filamentous (long filaments to short rods), polyhedral (vesicular and envelope-like), and pleomorphic (including those that are lemon, droplet, and ampule shaped) (Ackermann, 2007). The nucleic acid content of phage genomes is either DNA or RNA and both double and single stranded DNA and RNA phages have been identified. In addition, the size of the phage genome can range from under ten kilobases to several hundred kilobases.
Phages have evolved replication strategies that can be lytic, lysogenic (temperate), or chronic. Chronic replication results in the continual, non-lethal shedding of virions by protrusion through the membrane. All phages have common life-cycle stages of adsorption, DNA injection and replication, virion production, and release. Tailed phages mediate host cell lysis through the combined action of a holin, which perforates the membrane, and an endolysin (lysin), which hydrolyses cell wall peptidoglycan. Lytic phages are restricted to a life-cycle that results in the lysis of their host. Temperate phages have two possible life-cycles: lysis, or the recombination of their genome at a chromosomal attachment site using a phage-encoded integrase. Temperate phages are maintained within the host chromosome by transcriptional repressors that determine when the phage undergoes an infectious or lytic switch. The lytic switch occurs when conditions within their host promote excision. Excision usually proceeds during times of hardship when host health is threatened, either by physical stress or by chemical stress, such as antibiotics, ultraviolet (UV) light, or reactive oxygen species (Allen, et al., 2011; DeMarini & Lawrence, 1992; Little & Mount, 1982). Temperate phages provide key insights into the evolution of bacterial pathogenesis, since many temperate phages encode virulence factors used by pathogenic bacteria during both human and animal infections (Bensing, Siboo, & Sullam, 2001; Brüssow, Canchaya, & Hardt, 2004; Novick, Christie, & Penadés, 2010).
Distribution of phages across the enterococci
The first documented reports of enterococcal phages were published over 70 years ago (Clark & Clark, 1927; Evans, 1934). It was not until the early 1960s that more comprehensive analyses of enterococcal phages began to take shape (Brock, 1964; Rogers & Sarles, 1963). At the time, which was prior to the advent of modern molecular phylogenetics, the enterococci were characterized as group D streptococci. Rogers and Sarles (Rogers & Sarles, 1963) isolated phage-like particles from the intestinal tracts of Sprague-Dawley rats, and identified two phages that were capable of forming plaques on Streptococcus faecalis var. zymogenes. After careful analysis of host range using intestinal isolates of S. faecalis, followed by phage antibody serotyping, Rogers and Sarles captured some of the first images of enterococcal phages by using electron microscopy. They determined that the enterococcal phages appeared to have icosahedral heads and long non-contractile tails (Rogers & Sarles, 1963). One year later, Thomas Brock published a detailed survey of the host range of several enterococcal phages and identified enterococcal lysogens for the first time (Brock, 1964). Furthermore, Brock made the distinction that when testing for plaque formation on S. faecalis var. zymogenes, Streptococcus faecalis var. liquefaciens, and Streptococcus faecium, a degree of cross-reactivity of the phages for different host strains was present. Interestingly, this promiscuity was unique within S. faecalis and S. faecium, and phages specific to one species did not otherwise infect the other. When this type of phage resistance does occur, it is often due to inherent differences between species, with respect to their cell wall structure, mechanisms of protection against the acquisition of foreign DNA, and immunity due to lysogeny (superinfection exclusion).
Currently the well-studied enterococcal phages are those that infect and lysogenize Enterococcus faecalis and Enterococcus faecium. Numerous lytic phages that infect E. faecalis and E. faecium have been isolated from diverse environments, including sewage and wastewater sites, livestock runoff, and the intestinal tract (Horiuchi, Sakka, Hayashi, Shimada, Kimura, & Sakka, 2012; Lee & Park, 2012; Mazaheri Nezhad Fard, Barton, & Heuzenroeder, 2010; Otawa, Hirakata, Kaku, & Nakai, 2012; Parasion, Kwiatek, Mizak, Gryko, Bartoszcze, & Kocik, 2012; Rogers & Sarles, 1963). To achieve host lysis phages encode cell membrane holins and peptidoglycan hydrolases. These cell wall hydrolases, most commonly from lytic phages, have great potential as novel therapeutics that can target pathogenic strains of E. faecalis and E. faecium.
Known phage families found among Enterococci
Until recently, all characterized enterococcal phages belonged to the Podoviridae, Siphoviridae, or Myoviridae families. These phages exhibit considerable genetic and morphological diversity. For example, the genome sequence of E. faecalis strain 62, which was isolated from a healthy infant, revealed the presence of a Podoviridae phage, EF62Φ, which has a linear extrachromasomal genome. The maintenance of EF62Φ in E. faecalis is proposed to be the result of an encoded RepB and a toxin-antitoxin system (Brede, Snipen, Ussery, Nederbragt, & Nes, 2011). Mazaheri Nezhad Fard et al. isolated the first non-tailed enterococcal phages that belong to the polyhedral, filamentous, and pleomorphic (PFP) phages. In this study, the authors isolated phages from E. faecalis, E. faecium, and Enterococcus gallinarum strains found in piggery effluent. These phages, which were similar to PFPs, included a filamentous Inoviridae family phage, a polyhedral phage of the Leviviridae family, and several abnormally shaped pleomorphic phages that resembled droplet or lemon-like structures and which belong to the Guttaviridae and Fuselloviridae families (Mazaheri Nezhad Fard, Barton, & Heuzenroeder, 2010). Little is known about the genetic organization of these diverse phages, and it is also unclear whether these phages have lysogenic life-cycles.
Environments where enterococcal phages are found
Enterococcal species reside in the oral cavity and urogenital tract of mammals as well as the digestive systems of mammals and insects. Enterococci are also frequently found in fecal waste sites, such as sewage treatment plants and uncontained areas of fecal contaminated groundwater. Furthermore, enterococci have evolved to become opportunistic pathogens that cause life-threatening infections, including endocarditis, septic bacteremia, and hospital-acquired wound infections. It is quite likely that the phages associated with different enterococcal species are also located within these diverse environments.
Enterococcal phages have been isolated from human and animal origins where the enterococci live as commensal bacteria (Caprioli, Zaccour, & Kasatiya, 1975; Nigutová, Styriak, Javorský, & Pristas, 2008; Rogers & Sarles, 1963). A comprehensive study from the 1970s isolated numerous enterococcal phages from the human urogenital tract, including phages found at urethral, endocervical, and ano-rectal body sites (Caprioli, Zaccour, & Kasatiya, 1975). These phages were highly successful at infecting numerous E. faecalis and E. faecium strains. Based on their ability to infect and lyse these enterococci, the phages were used to classify isolates of E. faecalis and E. faecium into 27 and 22 distinct strain types, respectively (Caprioli, Zaccour, & Kasatiya, 1975). This study highlights the importance of environments where enterococci are commensal bacteria as sources of phages. Further studies of these types of phages both within and outside their natural habitats may shed light on the ecology and community dynamics of commensal enterococci.
Enterococcal phages isolated from the intestinal tracts of rodents have been characterized (Rogers & Sarles, 1963); however, studies on enterococcal phage particles from human intestinal contents are limited. One particular study that addresses the composition of intestinal bacterial communities associated with a premature low-weight infant discovered that this infant maintained an amplified clonal population of Enterococcus in its intestines (Morowitz, et al., 2010). It was determined that the 16S rRNA sequence of this enterococcal strain, UC1ENC, was identical to the 16S rRNA sequences of several E. faecalis strains. Using total bacterial DNA sequences from the infant gut, the authors were able to reassemble the UC1ENC genome. UC1ENC shared ~81% of its protein coding sequence with E. faecalis V583. Two UC1ENC chromosomal prophage elements shared DNA sequence similarity with two phages (phage02 and phage04) found in the E. faecalis V583 chromosome. A second study further substantiated this evidence, and showed that the rise of Enterococcus within premature low-weight infants was not exclusive to the individual infant of the Morowitz et al. study. These data showed that in the case of eleven different premature low-weight infants, 75% of the individuals screened were highly populated with Enterococcus species within their intestinal bacterial communities (LaTuga, et al., 2011). In this study, numerous phage DNA sequences were also identified from the intestinal contents of the premature low-weight infants; however, this analysis did not elaborate as to whether any of these phages may be associated with the colonizing enterococcal strains. As an effort to better understand the microbial communities of the intestinal tract, the National Institutes of Health–funded Human Microbiome Project has begun to sequence numerous bacterial isolates from the intestine, including many species of enterococci (Proctor, 2011). These enterococcal genome sequences have revealed a large number of putative prophages that may be important to the commensal biology of the enterococci in the intestine.
The enterococci are also commensals of the mammalian oral cavity, and in some instances, have been associated with periodontal disease (Kayaoglu & Ørstavik, 2004). Enterococcal infections of the root canal, especially those caused by E. faecalis, are extremely resistant to current therapies (Stuart, Schwartz, Beeson, & Owatz, 2006). Unlike the intestinal tract, E. faecalis phages from the human oral cavity have been isolated and studied in detail. E. faecalis isolates have been recovered from the infected root canals of humans for whom therapeutic interventions were unsuccessful (Stevens, Ektefaie, & Fouts, 2011; Stevens, Porras, & Delisle, 2009). Four out of ten of these E. faecalis isolates could be induced to produce lytic phages through mitomycin C treatment. Three of these phages resembled Siphoviridae phages, and were characterized by long non-contractile tails and spherical heads. The fourth phage identified resembled a phage that was more closely related to the Myoviridae, with a contractile tail with tail fibers and an icosahedral head structure. This study was the first to show that enterococcal strains living within the oral cavity can be induced to produce phages. Enterococcal phages have been used to successfully reduce the ability of E. faecalis to grow on the surface of human dental roots. A phage multiplicity of infection of 0.1 was sufficient to minimize the ability of E. faecalis to colonize dental roots (Paisano, Spira, Cai, & Bombana, 2004). Treatment of E. faecalis endontic infection is difficult and recurrent infection is a concern. It has been proposed that phages that infect these bacteria may prove useful as an alternative to current treatment options (Paisano, Spira, Cai, & Bombana, 2004; Stevens, Porras, & Delisle, 2009).
Being members of the intestinal microbiota, Enterococcus species are shed from humans and animals in fecal waste. Phages have been instrumental in determining the existence of enterococci within contaminated water environments and are currently being used to monitor fecal contaminated water sources for the presence of enterococcal strains. One method relies on Enterococcus isolates from diverse contaminated water sources including waste-water run-off areas of grazing cattle, pigs, or sheep, and municipal waste-water sites (Räisänen, et al., 2007). These enterococcal isolates, which include multiple strains from the species E. faecalis, E. faecium, E. gallinarum, and Enterococcus casseliflavus, are used to survey water samples where enterococcal contamination is unknown. Enterococcal contamination within these water samples is indicated by the presence of phages capable of infecting the collection of enterococcal strains. This method, referred to as microbial source tracking, relies on using indicator strains of enterococci to identify infectious phages within water samples as a metric of enterococcal fecal contamination (Purnell, Ebdon, & Tayor, 2011). A second method uses a similar approach; however, a bank of known enterococcal phages termed “enterophages” are used to screen water samples for fecal E. faecalis contamination (Bonilla, Santiago, Marcos, Urdaneta, Domingo, & Toranzos, 2010; Santiago-Rodriguez, et al., 2010). Enterophages exclusively infect strains of E. faecalis and are present at levels of ~102 phages per 100 ml of domestic raw sewage (Bonilla, Santiago, Marcos, Urdaneta, Domingo, & Toranzos, 2010). Enterophages are diverse and include phages similar to the Siphoviridae as well as non-tailed phages with icosahedral shaped capsids. It is also thought that enterophages may be indicative of water sources contaminated with human fecal waste, although, enterophages similar to those isolated from contaminated water sources have yet to be identified in human stool samples (Santiago-Rodriguez, et al., 2010).
Enterococcal Temperate Phages and the Impact of Lysogeny
Temperate phages possess an alternative life-cycle that is absent from the reproduction of lytic bacteriophages, whereby the bacterial host harbors the phage genome and then replicates it during cell division. This transmits the phage genome vertically to daughter cells, which subsequently propagate the phage. Lysogens—bacteria acting as phage genome hosts—express resistance to superinfection by the same phage, but not to superinfection by heterologous phages (Birge, 1994). Multiple lysogenic infections, over time and with heterologous temperate bacteriophages, produce polylysogens. Comparative genome analyses of several low G+C genera of the Bacilli subbranch, such as Enterococcus, Streptococcus, Staphylococcus, and Listeria reveal that polylysogeny is common. During lysogeny, which follows recombination of the phage genome into the host chromosome; most of the phage genes are repressed. Those genes that are expressed are mostly involved in the maintenance of lysogeny, and the expressed proteins have regulatory functions that prevent the transcription of genes encoding replication, morphogenesis, and lytic components responsible for the lytic life-cycle of the phage (Ptashne, 2004). Phage conversion genes that are present on some prophages are often expressed during lysogeny. In numerous prophages of the low G+C Firmicutes (e.g. Staphylococcus aureus), phage conversion genes encode known or proposed virulence and fitness genes (Desiere, Lucchini, Canchaya, Ventura, & Brüssow, 2002; Prévost, et al., 1995; Tormo, et al., 2008; van Wamel, Rooijakkers, Ruyken, van Kessel, & van Strijp, 2006). Lysogeny is not a permanent state and during bacterial growth infective virions arise due to spontaneous prophage induction. The rate at which prophages enter the lytic cycle is phage and host-specific and, in addition, chemical or physical agents that damage DNA, including oxidants, some antibiotics like mitomycin C, and UV radiation, can all induce prophage entry into the lytic cycle.
Temperate phage genomics
The 3.2 Mb chromosome of the vancomycin-resistant E. faecalis strain V583 revealed that lysogeny contributed the largest component of horizontally-acquired DNA in this clinical isolate. Seven potential integrated phage–derived sequences were identified that comprised close to 10% of the host cell DNA (Paulsen, et al., 2003). phage01 (EF0303-55), phage03 (EF1417-89), phage04 (EF1988-2043), phage05 (EF2084-145), and phage06 (EF2798-855) have sufficient composition for integration/excision, DNA replication, and capsid/tail morphogenesis to generate functional virions, either alone or synergistically with other prophages. The phage02 region (EF1276-93) identified in the V583 genome, was later described to form part of the core genome (McBride, Fischetti, Leblanc, Moellering, Jr., & Gilmore, 2007). This region appears to be the remnant of a prophage and this cryptic phage likely retains no capacity for induction into the lytic life-cycle. Similarly, the phage07 region (EF2936-55) of strain V583 appeared to be a cryptic prophage but was recently shown to produce infectious virions (Duerkop, Clements, Rollins, Rodrigues, & Hooper, 2012; Matos, et al., 2013). Phage07 is similar to the phage-related chromosomal islands of Gram-positive bacteria which are mobile elements that utilize the packaging and structural elements of a helper phage for dissemination (Novick, Christie, & Penadés, 2010). Phage07 hijacks phage01 particles in this manner upon excision from the chromosome and has been re-named E. faecalis chromosomal island of V583 (EfCIV583) (Matos, et al., 2013). Sequencing of the 2.8 Mb genome of strain OG1RF, the parent of which was originally isolated from the human oral cavity, provided a stark contrast to strain V583, since only one phage element was present (the cryptic phage02). Multiple sequenced E. faecalis genomes are deposited in publicly-available databases and the presence or absence of prophages across many of these different genomes has been determined by using DNA microarray-based comparative genomic hybridization and comparative genomic analyses (Lepage, et al., 2006; McBride, Fischetti, Leblanc, Moellering, Jr., & Gilmore, 2007; Solheim, Brekke, Snipen, Willems, Nes, & Brede, 2011). These studies confirm the variable presence of prophage elements integrated at six regions identified in the V583 genome (phage01, phages 03–06, and EfCIV583).
Lysogenic conversion
In addition to bacterial genome comparison approaches, studies have described the genomics of purified virions liberated by phage-generated lysis after induction (Stevens, Porras, & Delisle, 2009; Yasmin, et al., 2010). The benefit of these studies lies in their capacity to determine the infectious temperate phages present within discrete strain sets, as well as a description of the laterally transferred DNA sequences. In the study by Yasmin et al. (Yasmin, et al., 2010), a collection of 47 clinical E. faecalis bacteremia isolates were screened for prophage induction using norfloxacin, mitomycin C, and UV light as stressors. Thirty-four unique phages were induced from these strains, as determined by host range and restriction fragment length polymorphisms (RFLP). Twelve strains in the study (26%) were polylysogens that contained up to five inducible prophages, based upon their host range and the restriction digest of DNA (Yasmin, et al., 2010). Of the phages that were identified, eight were confirmed as Siphoviridae by their morphology using electron microscopy indicating their long, non-contractile tails (~200 nm) and isometric capsids (~50 nm diameter) (Figure 1). The genome sequences of these phages were determined using DNA pyrosequencing.
Comparative genomic analyses grouped the eight Siphoviridae phages into four phage sequence types (ΦFL1A, B. and C; ΦFL2A and B; ΦFL3A and B; and ΦFL4A). Of these, ΦFL4A shares a high nucleotide identity with the phage01 region of strain V583, and the integrase proteins of these two phages have 99% amino acid identity (Yasmin, et al., 2010). The ΦFL1, ΦFL2, and ΦFL3 phages all have identical integrase proteins, and two of these phages, ΦFL2B and ΦFL3A, were induced from the same polylysogen host. This finding indicates that polylysogenized genomes are likely to exhibit diversity in the order that phages lysogenize their host. The DNA replication and packaging regions of the ΦFL1 and ΦFL2 phages share sequence identity with the phage03 and phage05 regions of strain V583, but appear to be otherwise distinct (Yasmin, et al., 2010). The study of Stevens et al. (Stevens, Porras, & Delisle, 2009) identified a single Siphoviridae phage, ΦEF11, from the lysogen host strain TUSoD11, following induction with mitomycin C, that was morphologically similar with those described by Yasmin et al. (Yasmin, et al., 2010). From these two studies, it appears that the genome organization of the Siphoviridae phages of E. faecalis is similar to that of many of the Siphoviridae phages that infect low G+C Gram-positive bacteria (Stevens, Ektefaie, & Fouts, 2011; Yasmin, et al., 2010). Phage genomes have been described as being modular in their organization (Desiere, Lucchini, Canchaya, Ventura, & Brüssow, 2002; Hatfull, Cresawn, & Hendrix, 2008) and the genomes of the described E. faecalis Siphoviridae phages are similarly modular within the three transcriptional units (Figure 2). The first unit of the prophage (i.e., as it appears on the host chromosome) is the leftward-transcribed integrase/cI region for the maintenance of lysogeny. A large rightward-transcribed region encoding proteins for the lytic pathway, replication, packaging, head/tail morphogenesis, and lysis functions is followed by a variable leftward-transcribed region. This terminal leftward-transcribed region between the lysin and the right-hand phage attachment site in many temperate phages of the low G+C Gram-positive bacteria often contains phage conversion genes. For example, the genes located in these regions resemble those that encode innate immune evasion proteins of S. aureus (Desiere, Lucchini, Canchaya, Ventura, & Brüssow, 2002; Prévost, et al., 1995; Tormo, et al., 2008; van Wamel, Rooijakkers, Ruyken, van Kessel, & van Strijp, 2006).
Analysis of the ΦEF11 phage genome and the genomes of the ΦFL1, ΦFL2, ΦFL3, ΦFL4 phages identified several potential phage conversion genes, also called cargo (Stevens, Ektefaie, & Fouts, 2011; Yasmin, et al., 2010). The ΦEF11 phage appears to have an extended set of lysins immediately followed by three leftward-transcribed genes, two of which encode a putative membrane protein and a lysM domain-containing protein (Stevens, Ektefaie, & Fouts, 2011). Based on their predicted function, these proteins are likely to be located on the host cell surface, and if expressed during growth, represent bona fide phage conversion genes. These proteins could also be involved with lysogeny or immunity to phages—or, as proposed by Stevens et al (Stevens, Porras, & Delisle, 2009), they may facilitate host cell lysis. The ΦFL1, ΦFL2, and ΦFL3 phages contain one or more small open reading frames within their cargo regions that have inferred amino acid sequence identity with control proteins of streptococcal phages, which suggests a possible role in the maintenance of lysogeny (Yasmin, et al., 2010). The ΦFL4 phage encodes no potential phage conversion genes in the terminal region of its genome. Further studies remain to be performed to investigate the carriage of phage conversion genes in enterococcal temperate phages.
The initial description of the E. faecalis strain V583 genome proposed that phage04 contains a ferrochelatase-encoding gene (EF1989) that could function in heme biosynthesis (Paulsen, et al., 2003). Within phage04, the gene cspA (EF1991) encodes a cold shock family protein homologous to that of E. coli (Lee, Xie, Jiang, Etchegaray, Jones, & Inouye, 1994). CspA homologs are encoded within a prophage genome of the Lactococcus lactis strain ll1403 and the Streptococcus phage bIL312 genome. A second copy of cspA (EF0781), with a high-sequence identity to the cspA homolog on phage04, is found elsewhere in the genome of its strain V583 host. Comparative prophage analyses have revealed that there are clear similarities between many modular phage genes present in Enterococcus, Lactococcus, Streptococcus, and Staphylococcus species (Paulsen, et al., 2003; Stevens, Ektefaie, & Fouts, 2011; Villion, Chopin, Deveau, Ehrlich, Moineau, & Chopin, 2009; Yasmin, et al., 2010). For example, the ΦFL3A and ΦFL3B phages share 34% and 31% sequence identity, respectively, with prophages of L. lactis subsp. cremoris SK11 and MG1363 (Yasmin, et al., 2010). As described earlier in this chapter, these bacteria often reside together in similar host environments, foodstuffs, or fecal-contaminated water sources, which may potentiate the proximal lateral transfer of DNA by phages and other mobile DNA elements.
E. faecium temperate phages
Phages that infect E. faecium and E. faecium genome–encoded prophages have been described in several studies (Galloway-Peña, Roh, Latorre, Qin, & Murray, 2012; Mazaheri Nezhad Fard, Barton, & Heuzenroeder, 2010; van Schaik, et al., 2010). The ability to induce prophages from a group of genome-sequenced E. faecium strains has recently been demonstrated (van Schaik, et al., 2010). These induced prophages were all Siphoviridae, and were morphologically identical to prophages from E. faecalis. Within the genome of these seven prophages, 3-5% of the coding sequence was determined to originate from phage DNA. This suggests that the majority of the E. faecium prophage sequence contributes potentially novel DNA, which drives the genomic diversity of these strains (van Schaik, et al., 2010).
Other enterococcal temperate phages
Far less is known about the temperate phages of other enterococci; however, current sequencing efforts will facilitate future studies. Non-faecalis and non-faecium enterococcal species , including E. casseliflavus and E. gallinarum, respectively, are likely to carry prophage elements, as determined by the presence of phage integrase genes in genome sequences from those strains (Palmer, et al., 2010). The phage genome integrity, boundaries, and organization of these elements are unclear and await annotation. Moreover, induction experiments are required to determine whether potential prophages can be induced from these species to produce infectious phage particles. Recent studies have also identified a number of novel enterococcal phages from diverse environments that can infect E. gallinarum and E. casseliflavus (discussed above) (Mazaheri Nezhad Fard, Barton, & Heuzenroeder, 2010; Purnell, Ebdon, & Tayor, 2011).
Role of phages in virulence
Comparative genome analyses of the E. faecalis V583 strain and of the ΦFL1, ΦFL2, ΦFL3, and ΦFL4 groups of phages revealed that they encoded multiple homologs of the Streptococcus mitis phage M1 PblA platelet-binding protein (Bensing, Siboo, & Sullam, 2001; Mitchell & Sullam, 2009). The PblA and PblB phage tail proteins of S. mitis were clearly demonstrated to contribute to platelet adhesion via interactions with the α2-8-linked sialic acid residues on gangliosides of platelet membranes (Mitchell & Sullam, 2009). V583 strains harboring singly lysogenized pbl gene containing phages (phage01, 04, or 06) adhered to human platelet cells, whereas lysogenized strains carrying only phage03 and 05 or EfCIV583 alone were unable to bind platelets (Matos, et al., 2013). The contribution to phage encoded Pbl proteins during E. faeclais diseases such as endocarditis has not yet been tested.
There have been no systematic studies that characterize the role of prophages and cryptic phages in the pathogenesis of E. faecalis. A study by Yasmin et al. (Yasmin, et al., 2010) revealed that ΦFL3A and ΦFL3B phage lysogens of E. faecalis JH2-2 reduced the survival of Galleria mellonella caterpillars, as compared to their non-lysogen parent strain. No clear effects were observed in this model for lysogens of the other phages identified. However, this preliminary investigation, using only one insect model of infection, did not study lysogen survival within the Galleria caterpillars, or the rates of spontaneous lysis for the various lysogens during growth. Moreover, it will be important to determine the transcription and replication of each of the phage elements during growth in the infection models. In contrast to E. faecalis, no comparative genomic analysis of E. faecium phages has been undertaken to date, and as a result, their potential contribution to pathogenesis is therefore unknown. The sequential deletion of prophage elements from a lysogenized enterococcal chromosome, or the generation of allelic replacement mutants of phage genes prior to comparative virulence studies, represents a more rigorous approach for future analysis. These types of studies have been performed in S. aureus (Bae, Baba, Hiramatsu, & Schneewind, 2006) and E. coli (Wang, et al., 2010), and in each case, multiple phage-encoded genes were found to positively contribute to changes in either virulence potential or environmental survival (such as antibiotic resistance).
More recently, a comparative genomic analysis of hospital-associated clonal complex 2 (CC2) strains of E. faecalis revealed that there was enrichment of multiple lateral transfer elements in these strains (Solheim, Brekke, Snipen, Willems, Nes, & Brede, 2011). Among 252 genes that were statistically enriched in CC2, as compared to non-CC2 strains, the majority of such genes were found in known lateral transfer elements. This group included 51 genes (nearly the complete genome) of phage03 and several genes of phage04 (Solheim, Brekke, Snipen, Willems, Nes, & Brede, 2011). Most of these genes were expressed based upon transcription analyses; however, no account was recorded for spontaneous lysis from prophages entering the lytic cycle within the cultures, which could account for the observed transcription. Aside from this finding, the determination that phage elements are positively correlated with virulence enhancement supports the future study of phage deletion strains, as described above.
Phage transduction
Temperate phages are well-recognized for their lateral gene transfer capabilities. The bacteriophage-mediated transfer of DNA from a donor cell to a recipient cell is termed transduction, and can be mediated by either lytic or temperate phages. Transducing particles occur during the lytic stage of phage life-cycles and can be of two types: either generalized or specialized transducing phage particles. Generalized transducing particles arise from the packaging of any host bacterial genome sequence of the requisite size with the absence of phage DNA. Specialized transducing phage particles are generated only by a temperate phage that excise and package DNA immediately adjacent to one end of the integrated prophage through covalent attachment (Birge, 1994). Transduction has the capacity to facilitate lateral gene transfer and was demonstrated in the enterococci by using the lytic phage EFRM1 (discussed below).
It was recently shown that several E. faecalis temperate phages were capable of generalized transduction (Yasmin, et al., 2010). Temperate phage transduction in this study was demonstrated by the transfer and recombination of DNA encoding antibiotic resistance in E. faecalis. This termperate phage transduction property has implications for the evolution of multi-drug resistant enterococci, as well as being a generally useful tool for laboratory-mediated genetic transfer. The capacity of enterococcal phages to facilitate generalized transduction was extended in a later study, which proposed that phages could mediate inter-species transfer of antibiotic resistance genes between different enterococci, including E. faecalis, E. faecium, E. gallinarum, Enterococcus hirae, and E. casseliflavus (Mazaheri Nezhad Fard, Barton, & Heuzenroeder, 2011).
Enterococcal Lytic Phages
By definition, a lytic phage is one that can infect a bacterial host strain, but does not possess the ability to lysogenize. Lytic phages are considered predators of bacteria, as their purpose is to infect, replicate, and lyse the host for transmission. This purpose opposes to that of temperate phages, which can enter a life-cycle of parasitism maintaining chronic infection within their host (Weinbauer, 2004). Of course, prophages themselves can enter the lytic life-cycle under specific inducing conditions and at host strain-specific frequencies. Lytic phages are often devoid of an integrase gene and lack the genes for the maintenance of lysogeny, which is why these phages exist in a perpetually predatory state. Lytic phages and the enzymes that code for host cell wall destruction, including the membrane-permeating holins and peptidoglycan hydrolytic enzymes, called lysins, are debated as alternative therapies against bacteria resistant to multiple antibiotics (Chhibber, Kaur, & Kumari, 2008; Matsuzaki, et al., 2003; McVay, Velásquez, & Fralick, 2007). Several obligate lytic enterococcal phages have been identified (Horiuchi, Sakka, Hayashi, Shimada, Kimura, & Sakka, 2012; Lee & Park, 2012; Mazaheri Nezhad Fard, Barton, & Heuzenroeder, 2010; Otawa, Hirakata, Kaku, & Nakai, 2012; Parasion, Kwiatek, Mizak, Gryko, Bartoszcze, & Kocik, 2012; Parasion, Kwiatek, Mizak, Gryko, Bartoszcze, & Kocik, 2012); however, due to space restrictions only a few will be discussed in detail.
ϕEF24C
The lytic phage ϕEF24C was isolated from a water channel in Kochi City, Japan after passage in culture with E. faecalis strain EF24 (Uchiyama, et al., 2008). Further analysis revealed that ϕEF24C was able to infect and proliferate in multiple strains of E. faecalis, including several vancomycin-resistant isolates; however, ϕEF24C was unable to infect or lyse any of the ten E. faecium strains that were tested. ϕEF24C shows broad activity for E. faecalis, but appears to lack the ability of cross-strain infectivity and lysis. The phylogeny of ϕEF24C places this phage in the Myoviridae family. ϕEF24C has an icosahedral head of ~93 nm in diameter and a contractile tail that when fully extended is ~204 nm in length (Uchiyama, et al., 2008). N-terminal degradation sequencing of many of the ϕEF24C structural proteins, including tail and capsid proteins, suggests that ϕEF24C is related to the SPO1 phage genus, which includes members such as Staphylococcus phages K and 812, Lactobacillus phage LP65, and Listeria phage P100, all of which are known lytic phages (Chibani-Chennoufi, Dillmann, Marvin-Guy, Rami-Shojaei, & Brüssow, 2004; Uchiyama, et al., 2008). The genome of ϕEF24C is large, with ~142 kbp of circular double stranded DNA. The ϕEF24C genome houses 221 open reading frames and five tRNA synthetase genes (Uchiyama, Rashel, Takemura, Wakiguchi, & Matsuzaki, 2008). Just fewer than 50% of the open reading frames found in the ϕEF24C are predicted to encode proteins with known functional domains. The ϕEF24C genome is organized into three distinct modules. The first module is structural and includes the genes involved in capsid and tail biosynthesis. The second module contains genes that encode replication proteins. The third module is a short structural module that includes a gene with an immunoglobulin protein motif, which is thought to be structurally associated with the phage particle. Outside of these modular domains are genes that encode putative nucleic acid precursor biosynthesis enzymes, which may be involved in the de novo synthesis of modified nucleotides. Furthermore, the ϕEF24C genome contains no genes homologous to a site specific integrase, which verifies the strictly lytic life-cycle of ϕEF24C (Uchiyama, Rashel, Takemura, Wakiguchi, & Matsuzaki, 2008).
EFRM31
EFRM31 is a double-stranded DNA phage that was originally isolated from runoff water from a pig farm, and was amplified in E. faecalis isolates found within piggery waste water (Mazaheri Nezhad Fard, Barton, & Heuzenroeder, 2010). Electron microscopy revealed that EFRM31 is a member of the Siphoviridae family and has a long non-contractile tail (206 nm, length) and a spherical capsid (55 nm, width). The EFRM31 genome is a circular ~17 kbp genome and is characterized by low G+C content. There are 87 open reading frames that are predicted to encode proteins, and 17 of these open reading frames are putative morphogenesis genes that encode structural and accessory polypeptides for tail and capsid assembly. EFRM31 also encodes numerous proteins of unknown function (Fard, Barton, Arthur, & Heuzenroeder, 2010). The EFRM31 genome is homologous to the EFAP-1 bacteriophage genome, a lytic phage of E. faecalis (Son, et al., 2010).
Outside of encoding proteins for phage particle biosynthesis and phage genome replication, EFRM31 also encodes a putative virulence factor homologous to a zinc metalloprotease, a DNA primase gene, an antibiotic resistance membrane pump, and a polyamine transporter. These genes are clustered together and reside outside of the organized modules for phage particle assembly and genome replication. It is likely that these genes have been acquired from a bacterial host chromosome through recombination (Fard, Barton, Arthur, & Heuzenroeder, 2010). EFRM31 is proficient in packaging DNA, aside from its own chromosome, during phage particle assembly. This can be concluded from studies that measured the ability of EFRM31 to transduce antibiotic resistance markers from E. faecalis host strain chromosomal or plasmid DNA to enterococcal host strains where sensitivity to a particular antibiotic had been previously determined (Mazaheri Nezhad Fard, Barton, & Heuzenroeder, 2011). EFRM31 was highly capable of transducing gentamicin resistance to multiple enterococcal species, and as a result, EFRM31 was also able to undergo inter-species transduction of gentamicin resistance to two E. faecium strains and an Enterococcus isolate most closely related to the durans/hirae species (Mazaheri Nezhad Fard, Barton, & Heuzenroeder, 2011). In some, but not all, of these transfer events, there was an association of an ant2-I gene (which codes for an aminoglycoside nucleotidyltransferase) cassette acquisition that is known to confer low level gentamicin resistance in the transduced strain. Since transducing phages are mostly species-specific, EFRM31 is the first example of an enterococcal phage that is capable of inter-species host range generalized transduction.
EFAP-1
EFAP-1 is a lytic phage that was isolated from environmental samples taken from a variety of sources within a cowshed. EFAP-1 infects E. faecalis. This phage is a member of the Siphoviridae family, and by electron microscopy, was determined to have a non-contractile tail and an isometric capsid. EFAP-1 is most closely related to Bacillus phage ϕ105 and Staphylococcus prophage ϕPV83 (Son, et al., 2010). The EFAP-1 genome is composed of 24 open reading frames and similar to other related phages, the open reading frames are modular and consist of clusters of genes annotated to be involved in phage structure, host cell lysis, phage genome replication, and electron transport. Several open reading frames within these modules share homology with proteins that are found on bacterial chromosomes. Although these open reading frames may originate from bacterial chromosomes, they may perform functions for the EFAP-1 phage. For example, the gene ORF1 encodes a putative thioredoxin-like superfamily protein that may be involved in phage DNA replication and may also be used during bacterial cell electron transport (Son, et al., 2010). Several open reading frames found in the EFAP-1 genome show no sequence similarity to those found in the current public sequence databases, and as a result, have been delineated as genes of unknown function. This result suggests that, like many other phages, EFAP-1 encodes potentially novel genes that, if expressed, may uniquely aid in determining the biology of this lytic phage.
Many more enterococcal lytic phages have been identified including at least one that is known to infect E. faecium as well as several recently identified environmental phages of diverse morphologies that infect E. faecalis (Mazaheri Nezhad Fard, Barton, & Heuzenroeder, 2010; Otawa, Hirakata, Kaku, & Nakai, 2012).
Enterococcal phage lysins and therapeutic applications
After replication and packaging of phage particles within a bacterial cell, lytic phages must exit the host cell in order to disseminate to a new host bacterium. To achieve such an exodus, non-filamentous double stranded DNA phage genomes encode enzymes, typically referred to as lysins, that accumulate in the cytoplasm of the host cell and target the destruction of its cell wall (Fischetti, 2005). Lysins act on the peptidoglycan of the bacterial cell wall by binding to polysaccharide or peptide moieties and subsequently cleaving these macromolecules, which aids in the release of phage progeny. Lysins are diverse hydrolases, including endo-β-N-acetylglucosaminidase or N-acetylmuramidase, that bind to sugar residues of peptidoglycan and endopeptidases that target a peptide ligand of the peptidoglycan cell wall. A fourth type of lysin, N-acetylmuramoyl-L-alanine amidase (amidase), hydrolyzes the amide bond between the peptide moiety and the sugar moiety of peptidoglycan (Young R. , 1992). The activity of a lysin is coupled to a second phage protein known as a holin—a transmembrane protein that inserts into the bacterial cell membrane causing disruption (Wang, Smith, & Young, 2000). Following holin insertion, the lysin can then traverse the cell membrane and gain access to the cell wall peptidoglycan to cause lysis. Holins are instrumental in allowing lysins to target the cell wall, because most lysins lack N-terminal signal sequences and cannot independently gain access to the cell wall (Young, Wang, & Roof, 2000).
It has been postulated that phage lysins could be developed into an alternative to antimicrobials, as there is a great need for new antimicrobial agents to fight the steady rise in multi-drug resistant bacteria that has taken place in recent decades. Many phage lysins have been purified and these enzymes have been used in proof of principal experiments to kill pathogenic bacteria both in vitro and in vivo (Fischetti, 2005; Fischetti, 2003). Lysins as therapeutics have mostly been applied to Gram-positive bacteria, where the lysin has easy access to the peptidoglycan on the outside of the bacterial cell. This process is in direct contrast to Gram-negative bacteria, which usually resist killing by exogenous lysins, due to the peptidoglycan of these cells being protected by the bacterial outer membrane. The enterococci are notorious for their antimicrobial resistance phenotypes (Palmer, Kos, & Gilmore, 2010; Willems & Bonten, 2007). In some cases, enterococcal strains can be resistant to a broad range of antibiotics, including some recently developed antibiotics (Arias, et al., 2011; Palmer, Daniel, Hardy, Silverman, & Gilmore, 2011). Due to their intrinsic antibiotic resistance, the efficacy of enterococcal phage lysins as antimicrobial agents has been tested in various studies.
PlyV12 is a lysin that was identified from a genomic DNA library of the ϕ1 lytic Myoviridae family enterococcal phage. This DNA library was screened for lytic factors that had killing activity against the E. faecalis strain V12 found in the human urogenital tract (Caprioli, Zaccour, & Kasatiya, 1975; Yoong, Schuch, Nelson, & Fischetti, 2004). The identification of a bacteriolytic polypeptide, PlyV12, that shares homology with N-acetylmuramoyl-L-alanine amidase type lysin enzymes, had a high specific lytic activity against both E. faecalis and E. faecium strains, including vancomycin-resistant strains. Interestingly, PlyV12 was also active in killing strains of S. aureus, Staphylococcus epidermidis, and multiple groups of streptococci. This established the PlyV12 lysin as a broad spectrum bacteriolysin, which is uncharacteristic of these types of enzymes, as most kill only the species that the parental phage can infect (Fischetti, 2005). Furthermore, it is proposed that PlyV12 may target a cell wall moiety that is conserved within many Gram-positive genera. The amino acid sequence of PlyV12 revealed a protein of ~32 kDa that has an N-terminal catalytic domain, and that shares sequence similarity to N-acetylmuramoyl-L-alanine amidase -like lysins from the group A and B streptococci Streptococcus pyogenes and Streptococcus agalactiae, as well as Streptococcus pneumoniae and Streptococcus mitis (Yoong, Schuch, Nelson, & Fischetti, 2004). This similarity in the lysin catalytic domain may account for the broad-spectrum killing ability of PlyV12, although the C-terminal region of PlyV12 where the predicted lysin epitope binding site resides is highly divergent from these streptococcal lysins (Yoong, Schuch, Nelson, & Fischetti, 2004).
A second study focused on a lysin enzyme from the lytic phage EFAP-1. This lysin, designated as EFAL-1, is also predicted to be a cell wall hydrolytic N-acetylmuramoyl-L-alanine amidase. EFAL-1 was purified after overexpression in E. coli cells, and was found to have broad spectrum killing activity (Son, et al., 2010). EFAL-1 was found to be lytic against 13 E. faecalis strains, seven E. faecium strains, two strains of S. agalactiae, and two strains of Streptococcus uberis. This potent activity of EFAL-1 is impressive, considering the parental lytic phage that encodes this lysin, EFAP-1, is only capable of lysing a single E. faecalis strain from this group of susceptible bacteria.
A third study characterized the lysin encoded by orf9 from the lytic enterococcal phage ϕEF24C. Using MALDI-TOF mass spectrometry, Orf9 was shown to specifically cleave peptidoglycan at the peptide sugar linkage, which confirms that Orf9 is also a hydrolytic N-acetylmuramoyl-L-alanine amidase (Uchiyama, et al., 2011). Site-specific truncations of the orf9 coding region confirmed that the ability of Orf9 to lyse E. faecalis depended on an intact C-terminus. An extensive analysis of the N-terminal region of Orf9 was not possible, as truncations within this region rendered the protein insoluble (Uchiyama, et al., 2011). Unlike the EFAL-1 and PlyV12 lysins, Orf9 was shown to have lytic activity against only E. faecalis and E. faecium. However, this analysis was only extended to 10 Staphylococcus strains, and further screening of other Gram-positive bacteria may reveal similar broad activity.
It is worth noting that phage lysins from non-enterococcal bacteria have been shown to be effective at killing Enterococcus species. The temperate phage ϕ-0303 of Lactobacillus helveticus encodes a 40 kDa lysin called Mur-LH that functions as a cell wall–degrading N-acetylmuramidase (Deutsch, Guezenec, Piot, Foster, & Lortal, 2004). Purified Mur-LH can lyse a diverse array of Gram-positive bacteria, including E. faecium. Mur-LH was unable to lyse E. faecalis, suggesting that Mur-LH binds to a cell surface epitope that is not shared between these two enterococcal species. Lactobacillus gasseri phage ϕgaY encodes an N-acetylmuramidase. This lysin, LysgaY, exhibits broad bacteriolytic activity and can lyse over 20 different species of Gram-positive bacteria, including enterococci (Sugahara, et al., 2007). Purified LysgaY also causes logarithmically growing Gram-positive bacterial cells to aggregate and form long chains. This phenotype is mostly likely caused by LysgaY binding to the site of cell wall peptidoglycan synthesis during cell division, which results in the inability of daughter cells to completely partition.
These data emphasize two important points. First, contrary to popular belief, many lysins, specifically those of enterococcal lytic phages, have broad tropism and are capable of intra- and inter-generic killing. Second, bactericidal therapeutics based on highly promiscuous lysins may be powerful tools for combating diverse Gram-positive pathogens. Lysins have been shown to act synergistically with some antibiotics (Loeffler & Fischetti, 2003) and specifically when used in combination with antibiotics that target cell wall synthesis, like penicillin. Bacterial resistance to lysins appears to be a rare event. It is thought that low incidence resistance to phage lysins is caused by phages evolving their lysins to target essential molecules in the bacterial cell wall (Fischetti, 2008). Therapies that combine phage lysins and antibiotics may also decrease the incidence of bacterial resistance through synergistic effects. However, one concern with this course of action is that phage lysins are enzymes, and therapeutic treatments using these types of proteins would result in the production of neutralizing antibodies and the elimination of the lysins (Fischetti, 2005). The efficacy of the bacteriolytic activity of lysins in the presence of neutralizing antibodies has been addressed. A study by Loeffler et al showed that a streptococcal phage lysin still maintained killing in the presence of specific neutralizing antibodies, although it was reduced in activity (Loeffler, Djurkovic, & Fischetti, 2003). Furthermore, mice pre-immunized with a streptococcal lysin prior to receiving an intranasal S. pneumoniae challenge produced lysin-specific neutralizing antibodies, yet were still protected upon administration of the lysin following infection (Loeffler, Djurkovic, & Fischetti, 2003). These data suggest that immunogenic phage lysins are still capable of acting against their target in the presence of neutralizing antibodies, and may warrant consideration for development into alternative antimicrobial agents.
Enterococcal Genome Defense Mechanisms
Prokaryotes possess multiple mechanisms that can interfere with phage infection, including receptor/adsorption blocking; abortive infection; clustered, regularly interspaced short palindromic repeats (CRISPR) with CRISPR-associated (Cas) proteins (referred to here as CRISPR-Cas); and restriction modification (RM) (51). CRISPR-Cas and RM can also limit uptake of other mobile elements, such as plasmids (discussed below). Despite clear differences in the abundance of prophages, plasmids, and other mobile elements in enterococcal genomes (Bourgogne, et al., 2008; Leavis, Willems, van Wamel, Schuren, Caspers, & Bonten, 2007; Palmer, et al., 2012; Solheim, Brekke, Snipen, Willems, Nes, & Brede, 2011), little is known about the mechanisms by which some strains appear more susceptible to phage infection and plasmid uptake than others. Recent studies have highlighted potential roles for genome defense systems in the evolution of multidrug resistance and hospital adaptation in the enterococci (Lindenstrauss, Pavlovic, Bringmann, Behr, Ehrmann, & Vogel, 2011; Palmer & Gilmore, 2010; Solheim, Brekke, Snipen, Willems, Nes, & Brede, 2011) and in the transfer of antibiotic resistance genes from enterococci to S. aureus (Monk, Shah, Xu, Tan, & Foster, 2012). This section introduces CRISPR-Cas proteins and restriction modification defense, and reviews evidence for these systems in the enterococci. Methods to identify CRISPR-cas loci in newly sequenced enterococcal genomes are also discussed. Because no phage receptors have been identified for the enterococci, nor have any phage abortive infection mechanisms been reported, those genome defense strategies are not discussed here.
CRISPR-Cas, a prokaryotic acquired immune system
Clusters of roughly palindromic repeat sequences, referred to as CRISPR, were identified in bacterial and archaeal genomes to be sequenced (Jansen, Embden, Gasstra, & Schouls, 2002; Mojica F. J., Díez-Villaseñor, Soria, & Juez, 2000). The repeats, which can vary in length (typically ~24-48 bp) and palindromic structure (Haft, Selengut, Mongodin, & Nelson, 2005; Kunin, Sorek, & Hugenholtz, 2007), were detected in 83% of archaeal and 56% of bacterial species analyzed by the CRISPI database as of February 2012 (103 archaeal and 956 bacterial species with available genome data) (Rousseau, Gonnet, Le Romancer, & Nicolas, 2009). Thus, CRISPR appear to be both ancient and widespread among prokaryotes. Genes encoding nucleases and other proteins involved in DNA and RNA processing are typically associated with CRISPR, and are called CRISPR-associated genes (cas genes) (Jansen, Embden, Gasstra, & Schouls, 2002). The cas genes encode Cas proteins. CRISPR-cas loci have diverse cas gene cohorts and repeat structures, and several classification systems have been reported (Haft, Selengut, Mongodin, & Nelson, 2005; Kunin, Sorek, & Hugenholtz, 2007; Makarova, Grishin, Shabalina, Wolf, & Koonin, 2006; Makarova, et al., 2011). Recently, a unifying nomenclature was established that groups CRISPR-Cas systems into three broad types (I, II, and III) defined by type-specific cas genes (Makarova, et al., 2011). Only two conserved cas genes, cas1 and cas2, are associated with all CRISPR-cas loci that are predicted to be functional (Makarova, et al., 2011). Exemplifying the diversity and complexity of these loci, the three broad CRISPR-Cas types function by distinct molecular mechanisms (Wiedenheft, Sternberg, & Doudna, 2012), and prokaryotes can harbor multiple CRISPR-cas loci of varying types in their genomes. The CRISPR-Cas type that appears to be most relevant to the enterococci is Type II, and this chapter focuses mainly on Type II systems. Readers should refer to an excellent and recent review for more information on Type I and Type III systems (Wiedenheft, Sternberg, & Doudna, 2012). Note that the Type II nomenclature was established in 2011 (Makarova, et al., 2011), and that some prior literature referred to these loci as Nmeni (Haft, Selengut, Mongodin, & Nelson, 2005) or Cas4 (Makarova, Grishin, Shabalina, Wolf, & Koonin, 2006) systems.
A representative Type II CRISPR-cas locus is shown in Figure 3A. Repeat sequences in Type II loci are typically 36 bp in length, and the unique sequences that occur between repeats, called spacers, are typically 30 bp (Haft, Selengut, Mongodin, & Nelson, 2005). The final repeat of the array is sometimes degenerate in sequence. A conserved leader sequence located 5ʹ to the repeat-spacer array drives the expression of the array (Deltcheva, et al., 2011) and likely contains motifs that are important for the addition of new spacers (Yosef, Goren, & Qimron, 2012). Type II CRISPR-cas loci typically possess 4 cas genes: the type-specific gene cas9, the core genes cas1 and cas2, and either csn2 or cas4 (Makarova, et al., 2011), although variations exist (Deltcheva, et al., 2011; Palmer & Gilmore, 2010).The csn2 gene appears to be specific to Type II-A systems, while cas4 has a wider distribution, being present in both Type I and Type II-B systems (Makarova, et al., 2011).
Catalytic mechanisms and crystal structures for some Cas proteins are known. Cas1 from the Type I CRISPR-Cas system of Pseudomonas aeruginosa is a metal-dependent deoxyribonuclease that generates 80 bp double-stranded DNA fragments (note that spacer lengths for this CRISPR-cas locus are 32 bp) (Wiedenheft, Zhou, Jinek, Coyle, Ma, & Doudna, 2009). Cas2 proteins from multiple prokaryotes have been characterized, and all are endoribonucleases that preferentially cleave single-stranded RNA targets in U-rich regions (Beloglazova, et al., 2008). Csn2 from E. faecalis ATCC 4200 has been purified and its structure characterized; the protein oligomerizes to form a tetrameric ring that binds double-stranded DNA (Nam, Kurinov, & Ke, 2011). The Type II-specific Cas9 proteins are large (~1000 amino acids) proteins that possess predicted RuvC-like nuclease and HNH restriction endonuclease-like domains (Makarova, Aravind, Wolf, & Koonin, 2011). No structural data are currently available for these proteins. Similarly, Cas4 is a predicted nuclease for which no structural data are available.
Genome analysis provided the initial evidence that CRISPR-Cas systems interact with mobile elements. Studies published in 2005 reported that some CRISPR spacers are identical to plasmid and phage sequences; a result that suggests that a prior encounter with mobile elements resulted in spacer acquisition (Figure 3B) (Bolotin, Quinquis, Sorokin, & Ehrlich, 2005; Mojica F. J., Díez-Villaseñor, García-Martínez, & Soria, 2005; Pourcel, Salvignol, & Vergnaud, 2005). A region of a phage, plasmid, or other genome with sequence identity to a spacer sequence is called a protospacer (Deveau, et al., 2008). A relationship between the presence of certain CRISPR spacers and the absence of prophage possessing corresponding protospacers was noted for S. pyogenes (Pourcel, Salvignol, & Vergnaud, 2005). These observations contributed to the hypothesis that spacer-derived small RNAs could block phage infection by interfering with phage gene expression via an RNA interference (RNAi)-like mechanism (Bolotin, Quinquis, Sorokin, & Ehrlich, 2005; Makarova, Grishin, Shabalina, Wolf, & Koonin, 2006; Mojica F. J., Díez-Villaseñor, García-Martínez, & Soria, 2005).
In 2007, a role for the Type II CRISPR-Cas as an anti-phage defense system of S. thermophilus was experimentally demonstrated (Barrangou, et al., 2007). The S. thermophilus type II CRISPR-cas locus possesses cas9, cas1, cas2, and cas4 genes, and for the purposes of this chapter, is referred to as StCRISPR1-Cas system. S. thermophilus DGCC7710 was challenged in vitro with lytic phages. The authors sequenced the StCRISPR1 of 9 phage-resistant mutants recovered from the phage challenge experiments and found that the loci had acquired new spacer sequences. The new spacer sequences were similar to coding and non-coding genomic sequences from the lytic phages used in the infection challenge. Novel spacer sequences were added only to the leader end of the CRISPR. Most notably, S. thermophilus mutant strains that were engineered to lack spacers became phage sensitive; while strains engineered to possess spacers matching phage sequence gained phage resistance.
These experiments confirmed that the newly acquired spacers contributed to phage resistance. One of two cas genes queried, cas9 (formerly cas5) (Makarova, et al., 2011) was also required for phage resistance. When taken together, these data demonstrated that the StCRISPR1-Cas system protected a subpopulation of S. thermophilus from phage attack through the acquisition of novel, heritable spacer sequences, which in turn provided protection from subsequent attacks by phages with similar sequences by a Cas9-dependent mechanism (Figure 3B and 3D). Additional work has found that StCRISPR1 can acquire spacers from a plasmid resident in S. thermophilus, which apparently promotes loss of that plasmid, also by a Cas9-dependent mechanism (Garneau, et al., 2010).
An additional Type II CRISPR-cas locus is present in S. thermophilus DGCC7710, called CRISPR3-Cas (referred to as StCRISPR3-Cas) (Horvath, et al., 2008). This locus possesses cas9, cas1, cas2, and csn2 genes, as well as a repeat sequence that is similar (but not identical) to the repeats of StCRISPR1 (Horvath, et al., 2008). Both StCRISPR1 and StCRISPR3 of S. thermophilus DGCC7710 obtain new spacers in response to phage challenges (Barrangou, et al., 2007; Horvath, et al., 2008); thus, each of these loci are active in S. thermophilus DGCC7710. Despite the presence and activity of StCRISPR3-Cas in S. thermophilus DGCC7710, that loci's cas9 is apparently unable to complement a StCRISPR1-Cas cas9 mutant (Barrangou, et al., 2007; Garneau, et al., 2010; Horvath, et al., 2008). This indicates that the Cas9 proteins are highly specific to certain repeat sequences and/or other CRISPR structures.
While spacer acquisition has been experimentally demonstrated for the Type II CRISPR-Cas system of S. thermophilus (Barrangou, et al., 2007; Deveau, et al., 2008; Garneau, et al., 2010), little of the mechanistic detail is known. It has been proposed that cas1 and cas2 are important for spacer addition regardless of CRISPR type, based on their biochemical activities and because of their ubiquity among CRISPR-cas loci (Makarova, Aravind, Wolf, & Koonin, 2011). In support of this theory, novel spacer addition occurs when both cas1 and cas2 are overexpressed in an E. coli strain that possesses a Type I CRISPR but otherwise lacks chromosomally encoded cas genes (Yosef, Goren, & Qimron, 2012). In the initial demonstration of StCRISPR1-Cas as an anti-phage system, the authors noted that spacer addition did not occur in strains in which the cas7 gene (now cas4) was insertionally inactivated (Barrangou, et al., 2007). A similar observation was made for anti-plasmid spacer acquisition experiments (Garneau, et al., 2010). Thus it appears that this gene may also play an important role in spacer acquisition.
The selection of novel spacer sequences does not appear to be random. Conserved sequence motifs have been identified immediately adjacent to protospacer sequences targeted by StCRISPR1-Cas (NNAGAAW) and STCRISPR3-Cas (NGGNG) (Deveau, et al., 2008; Horvath, et al., 2008), which suggests that these motifs play a role in spacer selection. In addition to a putative role in spacer selection, these protospacer-adjacent motifs (PAMs) (Mojica F. J., Díez-Villaseñor, García-Martínez, & Almendros, 2009) are important for the interference of StCRISPR-Cas with mobile elements. Mutations in the PAM allow both phages and plasmids to avoid Type II CRISPR interference (Deveau, et al., 2008; Garneau, et al., 2010; Sapranauskas, Gasiunas, Fremaux, Barrangou, Horvath, & Siksnys, 2011), although it appears that the StCRISPR1-Cas system tolerates non-consensus PAM sequences in plasmid, but not in phage targets (Garneau, et al., 2010). More work is required to determine the precise roles of PAMs in CRISPR spacer selection and mobile element interference, as well as why PAMs in the hostʹs own genome are not readily utilized for spacer selection.
What is the precise role of the CRISPR spacer in mobile element interference? It is now known that Type I, Type II, and Type III CRISPR repeat-spacer arrays are transcribed and processed to generate small guide RNAs called CRISPR RNAs (crRNAs) (Brouns, et al., 2008), which vary in structure depending on their CRISPR type (Wiedenheft, Sternberg, & Doudna, 2012). For Type II systems, transcription of the repeat-spacer array generates a long, pre-crRNA which is processed to short, 39-42 ribonucleotide (nt) crRNAs (Deltcheva, et al., 2011) (Figure 3C). This processing has been observed for Type II loci in S. thermophilus, S. pyogenes, Streptococcus mutans, and Listeria monocytogenes, among others (Deltcheva, et al., 2011). A mature Type II crRNA consists of 20 3ʹ nt of a spacer and 19-22 5ʹ nt of the following repeat. Thus, each Type II crRNA is a unique guide molecule that consists of a memory of a previous mobile element exposure (a partial spacer) and a conserved handle (a partial repeat) that likely associates with cellular machinery and prevents unproductive self-interference. Based on the structure of mature crRNAs, it appears that the 5ʹ end of the spacer sequence is less important than the 3ʹ end for crRNA-mediated interference. This is supported by the observation that when the Type II StCRISPR3-cas locus is heterologously expressed in E. coli, spacer-protospacer mismatches are tolerated at the 5ʹ spacer end and the central region (positions 2, 11, 18, and 23 of a 30 nt spacer), but not at the 3ʹ spacer end (positions 25 and 28) (Sapranauskas, Gasiunas, Fremaux, Barrangou, Horvath, & Siksnys, 2011). Additionally, StCRISPR1-Cas tolerates a spacer-plasmid protospacer mismatch at the first 5ʹ position of the spacer sequence (Garneau, et al., 2010).
Processing of the Type II pre-crRNA proceeds via a unique mechanism that is distinct from pre-crRNA processing in Type I and Type III systems (Deltcheva, et al., 2011; Wiedenheft, Sternberg, & Doudna, 2012). A second non-coding RNA associated with the Type II CRISPR-cas locus, called the trans-activating CRISPR RNA (tracrRNA), is required for pre-crRNA processing (Deltcheva, et al., 2011). For the type II CRISPR-cas locus of S. pyogenes, tracrRNA is encoded 5ʹ to csn1; however, the location of tracrRNA varies for different Type II loci. tracrRNA contains a repeat-like sequence oriented antisense to the repeats of pre-crRNA. tracrRNA acts as a guide in pre-crRNA processing and forms an RNA-RNA duplex with pre-crRNA repeats, thereby facilitating cleavage within the crRNA repeat/tracrRNA anti-repeat by the housekeeping RNase III. Interestingly, a role for RNase III in Type II crRNA maturation is reminiscent of small interfering RNA and microRNA processing in eukaryotes (Deltcheva, et al., 2011). Certain aspects of the Type II processing mechanism remain to be elucidated, including the factor that is responsible for cleavage within the spacer sequence to liberate a mature crRNA (Deltcheva, et al., 2011). While the cas9 gene is required for pre-crRNA and tracrRNA processing (Deltcheva, et al., 2011), the precise role of the Cas9 protein in processing is unknown. Cas9 may carry out cleavage within the spacer sequence to liberate a mature crRNA, and/or may stabilize and facilitate base pairing of pre-crRNA and tracrRNA (Deltcheva, et al., 2011). As expected based on their roles in pre-crRNA processing, RNase III, tracrRNA, and cas9 are each required for S. pyogenes CRISPR spacer-dependent interference with plasmid uptake (Deltcheva, et al., 2011).
There is evidence for crRNA-directed cleavage of both RNA and DNA targets in Type III CRISPR-Cas systems (Hale, et al., 2009; Marrafini & Sontheimer, 2008; Zhang, et al., 2012), but there is none thus far for Type II CRISPR-Cas systems. It is clear that DNA is a target of the StCRISPR1-Cas system (Garneau, et al., 2010). Blunt-end cleavage occurs within the target protospacer of double-stranded plasmid DNA, 3 bp 5ʹ to the PAM (after the 27th nucleotide of a 30 nucleotide protospacer) (Garneau, et al., 2010). The same cleavage site is observed for phage DNA targets (Garneau, et al., 2010). Cleavage occurs 3 bp 5ʹ to the PAM even if the spacer is < 30 bp, indicating that the cleavage proceeds by a 3ʹ anchored mechanism (Garneau, et al., 2010). An additional cleavage event occurs within protospacers when spacers target the positive strand of a phage genome. This second cleavage occurs within the protospacer, 19 or 20 bp 5ʹ to the PAM (35), which corresponds to the 5ʹ end of mature crRNAs (Deltcheva, et al., 2011). As a result, it appears that the Type II CRISPR-Cas interference machinery can discriminate between DNA strands in the target DNA. Because only a negative strand protospacer has been investigated for plasmid targets (Garneau, et al., 2010), it is unclear whether this strand discrimination is specific to phage targets or is a more general property of Type II CRISPR-Cas interference.
To summarize, Type II CRISPR-Cas are genome defense systems that evolve in response to plasmid and phage attacks and that confer immunity to progeny cells through a genetically programmed memory of these attacks (Figure 3). Despite rapid advances in this field, much remains to be learned about Type II CRISPR-Cas systems. Arguably the most important aspect are the mechanisms by which CRISPR-Cas exclude their own genome's potential spacers from inclusion in CRISPR arrays, and the precise roles of each of the Cas proteins in acquiring new spacers, processing crRNAs, and providing defense. While structural data are available for Type I (Wiedenheft, et al., 2011) and Type III (Zhang, et al., 2012) crRNA interference complexes (i.e. crRNA with its associated protein effectors), this is not the case for Type II. Determining a crystal structure for Cas9 will be especially informative, as this protein acts at both the crRNA processing and interference stages of Type II CRISPR defense. An open question remains as to why a 30 bp spacer is incorporated into a CRISPR array if only 2/3 of that sequence is used in a mature crRNA; perhaps different ruler mechanisms guide spacer addition and crRNA processing.
Finally, while many CRISPR spacers have sequence identity to plasmid and phage sequences, some have identity to the host's own genome These spacers are referred to as self-targeting spacers. Self-targeting spacers were identified in StCRISPR1 and StCRISPR3 loci of various S. thermophilus strains (Horvath, et al., 2008). The role of self-targeting spacers is unclear, although it has been hypothesized that CRISPR systems can erroneously incorporate "self" genome into the spacer array, leading to selection for progeny with degenerate (broken) CRISPR-cas loci (Stern, Keren, Wurtzel, Amitai, & Sorek, 2010). An alternative and not necessarily mutually exclusive explanation, depending on the CRISPR type in question, is that self-targeting spacers regulate the expression of chromosomal genes through an RNAi-like mechanism (Horvath, et al., 2008). Despite sequence identities of some CRISPR spacers to known mobile elements and host genomes, most spacers analyzed do not match known sequences—a finding that indicates that prokaryotes are exposed to a diverse variety of uncharacterized phages and plasmids that have not been sampled by genomic and metagenomic (total DNA retrieved from an environmental sample) studies to date.
Enterococcal CRISPR-Cas systems
Enterococcal CRISPR-cas was first identified in the E. faecalis OG1RF genome (Bourgogne, et al., 2008). A Type II-A locus possessing a CRISPR and cas9, cas1, cas2, and csn1 genes was identified in OG1RF between homologues of the E. faecalis V583 ORFs EF0672 and EF0673, which the authors named CRISPR1 (referred to as the EfsCRISPR1-cas locus for the purposes of this chapter, shown in Figure 4). An additional gene of unknown function is encoded 3ʹ to the CRISPR1 array in OG1RF. A second CRISPR locus that lacks cas genes was identified between V583 homologues of EF2062 and EF2063, which the authors named CRISPR2 (EfsCRISPR2 for the purposes of this chapter, and also shown in Figure 4). The consensus repeat sequences for EfsCRISPR1 and EfsCRISPR2 are identical, suggesting that the two loci are functionally linked (Bourgogne, et al., 2008; Horvath, Coûté-Monvoisin, Romero, Boyaval, Fremaux, & Barrangou, 2009). An EfsCRISPR2 locus of identical repeat sequence and different spacer content occurring between EF2062 and EF2063 was subsequently identified in the V583 genome (Horvath, Coûté-Monvoisin, Romero, Boyaval, Fremaux, & Barrangou, 2009; Palmer & Gilmore, 2010). The presence of EfsCRISPR1-cas in E. faecalis OG1RF was proposed to account for the low prophage content of this strain (OG1RF possesses only the cryptic phage02 which is part of the E. faecalis core genome) (Bourgogne, et al., 2008; McBride, Fischetti, Leblanc, Moellering, Jr., & Gilmore, 2007; Palmer, et al., 2012). This is in contrast to E. faecalis V583, which possesses seven prophage elements (Paulsen, et al., 2003) and lacks EfsCRISPR1-Cas (Bourgogne, et al., 2008). PCR-based screening for the presence of EfsCRISPR1, cas1, and csn1 in 14 additional E. faecalis isolates indicated that this locus is variable in the faecalis species (Bourgogne, et al., 2008).
The availability of genome sequence data for an additional 16 E. faecalis genomes (Palmer, et al., 2010) allowed for a more comprehensive analysis of CRISPR distribution in the faecalis species (Palmer & Gilmore, 2010). As expected, EfsCRISPR1-cas was variably distributed among the 16 genomes (present in 5/16), and when present occurred invariably between homologues of EF0672 and EF0673. The number of spacers in the loci varied, as did the presence of the gene of unknown function occurring 3ʹ to the CRISPR array in E. faecalis OG1RF. A second CRISPR-cas locus, named CRISPR3-cas (referred to as the EfsCRISPR3-cas locus for the purposes of this chapter), was identified in two additional faecalis genomes between homologues of the V583 ORFs EF1759 and EF1760 (Figure 4). EfsCRISPR3-cas encodes a putative Type II CRISPR-Cas system. Its cohort of cas genes contains csn1, cas1, cas2, and a gene of unknown function. EfsCRISPR3 repeats are distinct from EfsCRISPR1/CRISPR2 repeats, sharing only 42% nucleotide sequence identity. EfsCRISPR1-cas and EfsCRISPR3-cas loci did not co-occur in the same strain; thus, 7 of the 16 genomes analyzed possessed a Type II CRISPR-Cas system.
Interestingly, an EfsCRISPR2 locus of conserved repeat sequence and varying spacer content was identified between EF2063 and EF2061 homologues in all 16 E. faecalis genomes analyzed (Palmer & Gilmore, 2010). Note that EF2062 homologues are not annotated in these genomes, despite the nucleotide sequence being ≥ 98% conserved. As a result, it appears that an orphan CRISPR locus of varying spacer content is core to the faecalis genome, and in 9 of 16 genomes, this locus is maintained in the absence of cas genes. EfsCRISPR2 does not appear to confer defense from mobile elements, as genomes possessing only EfsCRISPR2 are significantly larger and encode more protein domains associated with mobile elements as compared to genomes possessing an EfsCRISPR1-cas or EfsCRISPR3-cas locus (Palmer, et al., 2012). Further, the absence of cas9 in EfsCRISPR2-only strains indicates that pre-crRNA generated from EfsCRISPR2 would not be processed to mature crRNAs.
Analysis of 140 spacer sequences extracted from the EfsCRISPR loci of 16 E. faecalis genomes revealed identities to phage and plasmid sequences (Palmer & Gilmore, 2010). It appears that the E. faecalis Type II CRISPR-Cas systems provide defense from attack by both lytic and temperate phage. Spacers in two strains were similar to sequences from the lytic enterococcal phage ΦEF24C (Uchiyama, et al., 2008), while spacers from seven strains were similar to sequences from the temperate phage ΦFL2B (Yasmin, et al., 2010), or prophages present in the V583 genome (Paulsen, et al., 2003). Spacers from six strains were similar to sequences from pheromone-responsive plasmids and plasmids integrated into the V583 genome, suggesting that E. faecalis Type II CRISPR-Cas systems also confer defense against these elements.
Because plasmids are common disseminators of antibiotic resistance genes in E. faecalis (Palmer, Kos, & Gilmore, 2010), it is likely that CRISPR-Cas acts as a barrier to the acquisition of these genes. This is supported by the finding that the absence of either Type II EfsCRISPR-cas locus is significantly associated with antibiotic resistance acquired by horizontal gene transfer in a collection of 48 E. faecalis strains (Palmer & Gilmore, 2010). Further, high-risk E. faecalis multilocus sequence typing (MLST) lineages lack EfsCRISPR1-cas and EfsCRISPR3-cas (Palmer & Gilmore, 2010), and the presence of certain virulence factor genes is associated with EfsCRISPR-cas absence in E. faecalis (Lindenstrauss, Pavlovic, Bringmann, Behr, Ehrmann, & Vogel, 2011). It appears that the absence of Type II CRISPR-Cas defense in certain enterococcal lineages explains the preponderance of acquired antibiotic resistance genes, prophage, plasmids, and other mobile element traits observed for these strains.
A Type II-A CRISPR-cas locus has also been identified in E. faecium, occurring in 3 of 8 draft genomes analyzed (Palmer & Gilmore, 2010). This locus, EfmCRISPR1-cas, possesses a CRISPR with repeat sequences that are distinct from the E. faecalis CRISPR loci, although EfmCRISPR1 appears to be more closely related to EfsCRISPR1 than EfsCRISPR1 is to EfsCRISPR3. EfmCRISPR1-cas possesses csn1, cas1, cas2, and csn2, as well as an additional gene of unknown function encoded 5ʹ to csn1. Spacers in one of the three strains are similar to predicted phage sequences from Clostridium novyi and L. lactis, and could be derived from an uncharacterized enterococcal phage. The EfmCRISPR-cas locus was absent from three vancomycin-resistant E. faecium strains and from three of four multidrug resistant strains, a finding that is consistent with a role for the system in limiting acquisition of antibiotic resistance genes by horizontal gene transfer.
Have Type II CRISPR-Cas systems been lost from certain E. faecalis and E. faecium lineages, and if so, by what mechanism? It was postulated that S. thermophilus Type II CRISPR-cas loci could be lost by recombination occurring between a CRISPR repeat and a partial repeat sequence occurring 5ʹ to the cas genes (likely the anti-repeat of tracrRNA (Deltcheva, et al., 2011; Horvath, et al., 2008). This implies the deletion of the CRISPR-cas locus, which does not appear to be the mechanism underlying the presence of variable Type II CRISPR-cas loci in E. faecium or E. faecalis. For E. faecium, genomic analyses have revealed that what is clinically classified as E. faecium is actually composed of two distinct phylogenetic clades (Galloway-Peña, Roh, Latorre, Qin, & Murray, 2012; Palmer, et al., 2012) that possess ~ 4-6% divergence in shared gene sequences (Palmer, et al., 2012). Hospital adaptation appears to have originated from only one of these clades, while the other clade consists of fecal commensal or otherwise non-hospital adapted strains. Recombination can occur between strains from these two clades, generating new strains with hybrid genomes (Palmer, et al., 2012). Strikingly, the EfmCRISPR1-cas locus has been identified only in strains from the non-hospital adapted clade, as well as in a hybrid strain that apparently acquired the locus by recombination (Palmer, et al., 2012). This suggests that the ancestral absence of CRISPR-Cas defense in one E. faecium clade facilitated the eventual emergence of acquired multidrug resistance and hospital adaptation specifically from that clade.
Unlike E. faecium, many E. faecalis strains are so closely related in core genome sequence that their relationships to each other cannot be cleanly resolved by whole genome analysis (Palmer, et al., 2012). It is therefore more difficult to postulate that ancestral E. faecalis was composed of distinct clades heterogeneous for CRISPR-Cas defense. In the place of the EfsCRISPR1-cas locus, strains such as E. faecalis V583 possess a novel, ~200 nucleotide sequence found only in strains that lack that locus (Palmer & Gilmore, 2010). Similarly, strains lacking EfsCRISPR3-cas possess a novel, ~50 nucleotide sequence found only in strains that lack that locus. The origin and possible function of these novel sequences is unclear. What is clear is that recombination between E. faecalis strains can cause the loss of EfsCRISPR1-Cas.
Displacement of the OG1RF EfsCRISPR1-cas locus by incoming V583 genomic DNA has been observed for in vitro conjugation experiments (Manson, Hancock, & Gilmore, 2010; Palmer & Gilmore, 2010). Pheromone-responsive plasmids resident in the V583 cell can integrate into the chromosome, and from there initiate plasmid transfer functions, ultimately mobilizing large regions of the V583 chromosome into OG1RF recipients (Manson, Hancock, & Gilmore, 2010). These high frequency recombination-like transfers generate transconjugant strains with hybrid V583-OG1RF genomes that lack EfsCRISPR1-cas and concomitantly possess acquired antibiotic resistance genes and other clinically relevant traits originating from V583 (Manson, Hancock, & Gilmore, 2010; Palmer & Gilmore, 2010). Conceivably, antibiotic resistant, CRISPR-cas deficient strains such as these hybrid strains, as well as high-risk strains such as V583, could be specifically selected for during antibiotic therapy, and could ultimately become the dominant enterococcal lineages in antibiotic-treated patients. Collectively, data from E. faecium and E. faecalis genome analysis and plasmid transfer experiments indicate that enterococcal CRISPR-cas loci can be both disseminated and lost via recombination.
Methods to identify CRISPR-cas loci in enterococcal genomes
Several methods are available to search for CRISPR and cas genes in enterococcal genomes. Because the chromosomal locations of EfsCRISPR1-cas, EfsCRISPR2, EfsCRISPR3-cas, and EfmCRISPR1-cas are conserved (Palmer & Gilmore, 2010), those locations can be specifically interrogated for the presence or absence of these loci, either by genomic analysis or by PCR-based screening. To identify novel CRISPR loci, repeat finder programs can be used. A web-based program, CRISPRFinder (Grissa, Vergnaud, & Pourcel, 2007), is useful for the identification of CRISPR candidates. A genome sequence, either draft or complete, can be loaded into the CRISPRFinder web server and analyzed for potential CRISPR content with a short (< 1 minute) turnaround time. Candidates can then be mapped back to the genome sequence and compared to the structure of known CRISPR loci. As a caveat to this approach, small CRISPR loci like EfsCRISPR2 (consisting of 1 repeat, 1 spacer, and a degenerate repeat) are not identified by CRISPRFinder, and require annotation by analysis of a conserved genomic location (Palmer & Gilmore, 2010). If present, cas genes may be identified in the vicinity of CRISPR candidates, and can be easily found using genome annotations or by searching for genes encoding conserved protein domains (for example, TIGRFAM domains) that are diagnostic for CRISPR-Cas systems (Makarova, et al., 2011).
To illustrate this approach, analysis of the sequenced genome of Enterococcus italicus DSM 15952 (GenBank accession number AEPV00000000) for potential CRISPR-cas loci using CRISPRFinder, identified three potential CRISPR arrays: two located on genome contig 74 and one located on contig 75. The CRISPR on contig 75 possesses a 36 nucleotide repeat sequence and 23 spacer sequences with an average size of 30 nucleotides, and is associated with a set of cas genes including csn1, cas1, cas2, and csn2. Thus, E. italicus possesses a Type II-A CRISPR-cas locus. The two CRISPR on contig 74 flank a set of cas genes that include cas1, cas2, cas10, csm2, csm3, csm4, csm5, csm6, and cas6. cas10 is the signature gene for Type III CRISPR-cas loci, and csm2 is the signature gene for Type III-A CRISPR-cas loci (Makarova, et al., 2011). It appears that in addition to a Type II-A CRISPR-cas locus, E. italicus also possesses a Type III-A CRISPR-cas locus. Whether both the Type II-A and Type III-A CRISPR-Cas systems are active and whether they have overlapping or specialized functions in E. italicus genome defense remains to be determined.
Restriction-modification (RM)
There is a small amount of evidence for RM occurring in enterococci. A Type II restriction endonuclease, SfaI, was purified from Streptococcus faecalis var. zymogenes strain TR (likely an E. faecalis isolate) (Wu, King, & Jay, 1978). The recognition sequence of SfaI is GGCC, and cleavage occurs between the G and C (Wu, King, & Jay, 1978). SfaI activity was not identified in other S. faecalis and S. faecium strains, and based on its variable presence, the authors postulated that the gene encoding SfaI was plasmid-based (Wu, King, & Jay, 1978). Two other S. faecalis enzymes, SfaGU and SfaNI, were reported in a survey of RM enzymes, published in 1982 (Roberts R. J., 1984). A RM system (M.SfeI and R.SfeI) encoded by a Streptococcus faecalis SE72 plasmid has also been characterized (Okhapkina, et al., 2002). Finally, a methyltransferase with Dam-like activity encoded by the enterococcal VanB-type vancomycin resistance transposon Tn1549 has been identified (Radlińska, Piekarowicz, Galimand, & Bujnicki, 2005). Available literature suggests that RM does occur in the enterococci, although the systems identified to date are associated with mobile elements or are otherwise strain-specific. It remains to be determined whether these systems act as barriers to the uptake of additional mobile elements.
New England Biolabs maintains a database of computationally predicted and biochemically verified RM proteins, called REBASE (Roberts, Vincze, Posfai, & Macelis, 2010). A search for "Enterococcus" in REBASE yields 147 hits (as of March 2012), encompassing putative restriction, modification, and specificity subunits encoded by enterococcal chromosomes, plasmids, transposons, and temperate phages, including ΦFL1, ΦFL2, and ΦFL3. The majority of these RM candidates (100/147) are predicted Type II system components (as compared to 29 hits for Type I, 2 hits for Type III, and 15 hits for Type IV). For some of these proteins, recognition and/or cleavage sites have been identified. In the future, it will be of interest to integrate biochemical data from REBASE with genome analyses to better understand the distribution of these systems across the genus, and how their distributions might relate to prophage abundance, acquired antibiotic resistance, and hospital adaptation.
Perspectives and Future Directions
Enterococcal phage receptors
The receptors utilized by enterococcal phages and the host cell ligands that these receptors bind are mostly uncharacterized. To better understand the biology of enterococcal phages, it will be essential to identify the receptors they use to interact with their host. Peptidoglycan, repeating glycan strands of N-acetyl-D-glucosamine and N-acetylmuramic acid linked to small tetra-peptides, as well as cell wall teichoic acids, covalently linked to peptidoglycan disaccharides, are likely phage binding site candidates (Osborn, 1969; Tipper & Strominger, 1968). Additional teichoic acids called lipoteichoic acids are also anchored in the phospholipid membrane of enterococci. Teichoic acids in the cell wall of S. aureus and Bacillus subtilis have been determined to be phage receptors (Lindberg, 1973) and for S. aureus, alterations in wall teichoic acids results in phage resistance (Wolin, Archibald, & Baddiley, 1966). Lipoteichoic acids of the Lactobacillus delbrueckii cell membrane are also bound by phages. Substitutions in the glycerol backbone of the lipoteichoic acid render L. delbrueckii phage incapable of binding to the host cell surface (Räisänen, et al., 2007). Enterococcal teichoic acid structures vary. E. faecium U0317 produces a wall teichoic acid polymer of 2-acetamido-2-deoxy-D-galactose, glycerol, and phosphate (Bychowska, et al., 2011), while both poly(glycerol-phosphate) and poly(ribitol-phosphate) wall teichoic acid polymers have been detected in E. hirae ATCC 9790 (Armstrong, Baddiley, Buchanan, Davison, Kelemen, & Neuhaus, 1959) E. faecalis produces a 1,3 poly(glycerol-phosphate) polymer attached to either D-alanine, kojibiose (α-D-glucopyranosyl-(1,2)-α-D-glucose), or 6,6′-di-alanyl-α-kojibiose (Hogendorf, Bos, Overkleeft, Codée, & Marel, 2010). The sugars and peptides that compose the cell wall teichoic acids and lipoteichoic acids of the enterococci are strong candidates for enterococcal phage attachment structures. Previous work has also shown that the extracts of enterococcal cell walls can inactivate staphylococcal phages, a finding that suggests that cell wall sugars and/or peptides may be natural ligands for enterococcal phage binding (Rakieten & Tiffany, 1938).
Surface proteins are also likely receptor candidates for enterococcal phages. Numerous types of cell surface proteins have been identified, mainly in E. coli, as receptors for phages. These cell surface proteins represent a diverse array of protein types and include peptidoglycan-associated proteins, membrane transport proteins or channel porins, enzymes, substrate receptors used to transport metabolites, and secretion systems (Rakhuba, Kolomiets, Dey, & Novik, 2010). Enterococcal genomes contain many genes whose products are thought to be associated with the bacterial cell surface (Palmer, et al., 2012; Paulsen, et al., 2003). For instance, the E. faecalis V583 genome sequence contains greater than 50 putative lipoproteins with predicted signal sequences that may be associated with the E. faecalis membrane; approximately 20 different carbohydrate utilization pathways which have identifiable membrane transport proteins; and a large number of virulence related genes that are predicted to be exposed at the bacterial cell surface (Paulsen, et al., 2003). Many of these proteins may be used by enterococcal phages for the transmission of their nucleic acid during infections.
Other cell surface structures may be used for enterococcal phage attachment to the host cell, including capsular polysaccharides and pili. Capsular polysaccharides are secreted from bacterial cells and deposited at the cell surface, creating a layer of carbohydrate slime that covers the cell. In some instances, polysaccharide capsules are protective against phage adsorption; however, other phages utilize cell surface capsular polysaccharide for initial adsorption, a process which is often reversible (Lindberg, 1973; Rakhuba, Kolomiets, Dey, & Novik, 2010). E. faecalis encodes two clusters of genes that are known to contribute to capsule biosynthesis: the epa and cps genes. The epa genes are thought to contribute to the production of a rhamnose containing polysaccharide capsule, whereas the cps gene cluster polysaccharides are composed of glucose and galactose and are sometimes referred to as diheteroglycan (Hancock & Gilmore, 2002; Theilacker, et al., 2011; Thurlow, Thomas, & Hancock, 2009). The production of the cps polysaccharides is variable in E. faecalis and is the basis of the group A-D serotyping.
Little is known about capsule biogenesis in other enterococcal species, although E. faecium, E. casseliflavus, and E. gallinarum possess a putative capsule biosynthesis system that is distinct from the cps system of E. faecalis and is more similar to the capsule biosynthesis system of S. pneumoniae (Palmer, et al., 2012). It is possible that enterococcal phages bind to these polysaccharides in a cell trophic manner, due to the variable possession and expression of the capsular polysaccharide synthesis loci between enterococcal strains. If so, this may be a useful way to determine host specificity for particular enterococcal phage groups.
Some phages have also evolved to bind to the bacterial cell surface through interactions with surface appendages, such as flagella and pili (Lindberg, 1973; Rakhuba, Kolomiets, Dey, & Novik, 2010). Enterococci such as E. gallinarum and E. casseliflavus are flagellated, and many species produce pili (Nallapareddy, et al., 2006; Sillanpää, Prakash, Nallapareddy, & Murray, 2009). Pili receptors for phage adsorption have been identified for pleomorphic-RNA–containing phages and filamentous phages (Rakhuba, Kolomiets, Dey, & Novik, 2010). The recent identification of numerous novel enterococcal phages belonging to the polyhedral, filamentous, and pleomorphic phage group suggests that pili could be cell surface targets for phage adsorption (Mazaheri Nezhad Fard, Barton, & Heuzenroeder, 2010). Future studies on phage receptors of enterococci will be needed to better understand the mechanisms used by enterococcal phages to interact with their hosts, and may yield insight into how these phages influence the community structures of enterococci within natural habitats.
Role of enterococcal phages in mammalian systems
Studies have begun to elucidate the human virome, which is the type and abundance of viral particles found at distinct anatomical sites where commensal bacteria reside, which includes the intestinal tract, oral cavity, and the lungs (Pride, et al., 2012; Reyes, et al., 2010; Willner, et al., 2009). It is clear that the areas of the human body colonized by commensal bacteria are highly populated with phages. It is also evident that these phage populations fluctuate when changes in bacterial community structure occur; for instance, when the human diet is altered (Minot, et al., 2011). So far, our knowledge of the identity of these phages is limited to metagenomic DNA libraries of phage DNA sequences (i.e. integrases and phage structural genes) and their comparison to known phage and prophage sequences that have been deposited in public nucleotide databases. From these data, it is possible to obtain an idea of the diversity of natural phage populations associated with humans, and, to an extent, determine some information on the relative abundance of certain types of phages (i.e. double- versus single-stranded DNA phages) (Reyes, et al., 2010).
Analysis of metagenomic DNA sequences from the intestinal tract has revealed sequences with similarity to enterococcal phages, specifically those of E. faecalis (Duerkop, Clements, Rollins, Rodrigues, & Hooper, 2012; Morowitz, et al., 2010). Studying enterococcal species in the intestinal tract, especially those that are polylysogenic, may provide critical information about the biological significance of enterococcal phages in the intestines. It is possible that phages that infect the enterococci may influence genetic exchange between Enterococcus species within the intestine. Phage-mediated exchange of DNA may be another mechanism that enterococcal species use for generating genetic variation to evade the immune system at the intestinal mucosa. Enterococcal phages are a likely source of novel genes. Therefore, phage-mediated transfer of genetic traits may result in the acquisition of genes that contribute to hypervariable phenotypic traits for immune evasion—or, conversely, phages may transfer virulence factors that aid in the transition of enterococci from commensals to pathogens. This type of phage-bacterial interaction could have far-reaching implications for the evolution of pathogenic enterococci, as multiple species have evolved to become successful opportunistic pathogens. Recently, Enterococcal phages have been proposed to play an ecological role in the intestine by aiding in niche competition. In the intestines of gnotobiotic mice, prophage01 and EfCIV583 aided E. faecalis V583 during competition with closely related E. faecalis strains (Duerkop, Clements, Rollins, Rodrigues, & Hooper, 2012). This suggests that prophage induction could be a means by which certain communities of enterococci compete with neighboring, genetically similar communities for nutrients in the intestine by restricting the invasion of neighboring enterococcal strains through phage-mediated killing.
The use of in vitro co-culture systems and mouse models of enterococcal intestinal colonization, including the use of gnotobiotic mice, will be instrumental toward further elucidating the genetic and ecological roles of enterococcal phages within the intestinal tract. Furthermore, these types of studies are not limited to the intestinal tract and can be applied to other environments where enterococci reside, including the oral and vaginal microbiota, as well as endocarditis and bacteremia infections. It is likely that enterococcal phages will profoundly influence enterococcal biology within a multitude of different environments where both commensal and pathogenic enterococci are found, ultimately leading to discoveries relevant to the ways in which commensal and pathogenic enterococci interact with their mammalian hosts.
Enterococcal CRISPR-Cas and RM Systems
Most sequence analysis of enterococcal CRISPR was reported in 2010 (Palmer & Gilmore, 2010), prior to the discovery of tracrRNA and the structure of mature Type II CRISPR crRNAs (Deltcheva, et al., 2011). A strict sequence identity cut-off was used to identify enterococcal protospacer-spacer sequence matches (27/30 identical residues required) (Palmer & Gilmore, 2010), and it is likely that potential targets of the enterococcal CRISPR-Cas systems were missed. Based on current literature on Type II CRISPR-Cas systems, it now appears that 20/30 identical resides at the 3ʹ spacer end would be a stringent cut-off for spacer analysis. While informative for identifying crRNA targets (especially for E. faecium, for which few were identified), additional protospacers would facilitate the identification of consensus PAM sequences for each of the three enterococcal CRISPR-Cas systems. PAM sequences will be important to consider in designing experimental systems interrogating the specific roles of enterococcal CRISPR and CRISPR-Cas in conferring defense against phages and plasmids.
One attribute that distinguishes enterococcal Type II systems from those studied in other bacteria is the EfsCRISPR2 of E. faecalis. It is unclear how prevalent orphan CRISPR loci are in other prokaryotes. An orphan pre-crRNA in L. monocytogenes EGD-e appears to stabilize a transcript that encodes iron acquisition proteins (Mandin, Repoila, Vergassola, Geissmann, & Cossart, 2007). This result suggests that EfsCRISPR2 may have a secondary function in E. faecalis. Whether EfsCRISPR2 is expressed and processed remains to be determined, although the absence of cas9 suggests that the generation of mature crRNAs will not occur. A long transcript encoded antisense to the V583 ORF EF2062 and EfsCRISPR2 region has also been detected, which may complicate the analysis of EfsCRISPR2 expression and processing (d’Hérouel, et al., 2011). The significance of this antisense transcript is unknown, although presumably it could contribute to processing and/or stability of the EfsCRISPR2 transcript.
Beyond the CRISPR-Cas defense, it will be important to determine the way in which differential genome modifications and restriction enzyme cohorts affects phage susceptibilities, as well as the frequency of horizontal gene transfer in the enterococci. Restriction modification appears to be a major barrier to gene transfer in S. aureus (Monk, Shah, Xu, Tan, & Foster, 2012), and the same may be true for the enterococci. Bioinformatic identification and analysis of candidate restriction-modification and abortive infection systems from available enterococcal genomes will be informative, as very little is known about genome defense in the enterococci. Some therapeutic approaches have already started to explore the use of CRISPR and RM systems (Marrafini & Sontheimer, 2008; Torres, Jaenecke, Timmis, García, & Díaz, 2000).
Concluding Remarks
Enterococcal genomes harbor a diversity of integrated prophage and other mobile elements. Some strains are also susceptible to infection by non-integrating lytic phages. The study of phages and mobile element biology has revealed several exciting areas of enterococcal research. First, enterococcal phage lytic proteins, the lysins, have prospects as novel therapeutics that can target antibiotic resistant strains of enterococci and other Gram-positive bacteria that resist broad-spectrum antibiotic treatment. However, not only lysins, but also CRISPR, RM systems, and bacteriophages have the potential to be used as modern therapeutics. Second, enterococcal phages have great potential as important mediators of genetic exchange through transduction during intra- and inter-species infections. It is these phage-host interactions, along with other mobile elements like plasmids, which facilitate the acquisition of novel traits (i.e. antibiotic resistance determinants and virulence factors). The attainment of genes from various mobile elements helps to uniquely distinguish clonal populations of enterococci. Prophages and plasmids are abundant in the enterococci, even in strains thought to live a strictly commensal lifestyle. Therefore, the contribution of phage and plasmids to the biology of enterococci may provide insight into what role, if any, these elements play in the transition of enterococci from commensals to pathogens. That being said, one idea has surfaced. Many enterococci have evolved mechanisms to protect their genomes from the assault of plasmids and phage by employing CRISPR-Cas regulatory systems. These systems restrict host genome acquisition of foreign mobile elements through their destruction. Notably, those enterococcal strains possessing one or more complete CRISPR-Cas system are parasitized less by phage and other mobile elements which include antibiotic resistance genes. Those strains that lack functional CRISPR-Cas systems retain many more foreign DNA elements. This dichotomy may be one way in which enterococci have evolved to become successful opportunistic pathogens. If true, the lack of CRISPR-Cas systems would represent a legitimate mechanism for the promotion of the commensal to pathogen transition in the enterococci. These studies on enterococcal phage biology, mobile elements, and CRISPR-Cas have just begun to scratch the surface of an emerging field, wide open for new discoveries.
References
- Ackermann HW. 5500 Phages examined in the electron microscope. Arch Virol. 2007;152(2):227–243. [PubMed: 17051420]
- Allen HK, Looft T, Bayles DO, Humphrey S, Levine UY, Alt D, et al. Antibiotics in feed induce prophages in swine fecal microbiomes. MBio. 2011;2(6):e00260–e00211. [PMC free article: PMC3225969] [PubMed: 22128350]
- Arias CA, Panesso D, McGrath DM, Qin X, Mojica MF, Miller C, et al. Genetic Basis for In Vivo Daptomycin Resistance in Enterococci. N Engl J Med. 2011;365:892–900. [PMC free article: PMC3205971] [PubMed: 21899450]
- Armstrong JJ, Baddiley J, Buchanan JG, Davison AL, Kelemen MV, Neuhaus FC. Composition of teichoic acids from a number of bacterial walls. Nature. 1959;184:247–248. [PubMed: 13794315]
- Bae T, Baba T, Hiramatsu K, Schneewind O. Prophages of Staphylococcus aureus Newman and their contribution to virulence. Mol Microbiol. 2006;62(4):1035–1047. [PubMed: 17078814]
- Barrangou R, Fremaux C, Deveau H, Richards M, Boyaval P, Moineau S, et al. CRISPR Provides Acquired Resistance Against Viruses in Prokaryotes. Science. 2007;315(5819):1709–1712. [PubMed: 17379808]
- Beloglazova N, Brown G, Zimmermann MD, Proudfoot M, Makarova KS, Kudritska M, et al. A novel family of sequence-specific endoribonucleases associated with the clustered regularly interspaced short palindromic repeats. J Biol Chem. 2008;283(29):20361–20371. [PMC free article: PMC2459268] [PubMed: 18482976]
- Bensing BA, Siboo IR, Sullam PM. Proteins PblA and PblB of Streptococcus mitis, which promote binding to human platelets, are encoded within a lysogenic bacteriophage. Infect Immun. 2001;69(10):6186–6192. [PMC free article: PMC98750] [PubMed: 11553559]
- Birge EA. (1994). Bacterial and Bacteriophage Genetics (3rd ed.). New York: Springer-Verlag.
- Bolotin A, Quinquis B, Sorokin A, Ehrlich SD. Clustered regularly interspaced short palindrome repeats (CRISPRs) have spacers of extrachromosomal origin. Microbiology. 2005;151(Pt 8):2551–2561. [PubMed: 16079334]
- Bonilla N, Santiago T, Marcos P, Urdaneta M, Domingo JS, Toranzos GA. Enterophages, a group of phages infecting Enterococcus faecalis, and their potential as alternate indicators of human faecal contamination. Water Sci Technol. 2010;61(2):293–300. [PubMed: 20107254]
- Bourgogne A, Garsin DA, Qin X, Singh KV, Sillanpää J, Yerrapragada S, et al. Large scale variation in Enterococcus faecalis illustrated by the genome analysis of strain OG1RF. Genome Biol. 2008;9(7):R110. [PMC free article: PMC2530867] [PubMed: 18611278]
- Brede DA, Snipen LG, Ussery DW, Nederbragt AJ, Nes IF. Complete genome sequence of the commensal Enterococcus faecalis 62, isolated from a healthy Norwegian infant. J Bacteriol. 2011;193(9):2377. [PMC free article: PMC3133060] [PubMed: 21398545]
- Brock TD. Host Range of Certain Virulent and Temperate Bacteriophages Attacking Group D Streptococci. J Bacteriol. 1964;88(1):165–171. [PMC free article: PMC277273] [PubMed: 14197882]
- Brouns SJ, Jore MM, Lundgren M, Westra ER, Slijkhuis RJ, Snijders AP, et al. Small CRISPR RNAs guide antiviral defense in prokaryotes. Science. 2008;321(5891):960–964. [PMC free article: PMC5898235] [PubMed: 18703739]
- Brüssow H, Canchaya C, Hardt WD. Phages and the evolution of bacterial pathogens: from genomic rearrangements to lysogenic conversion. Microbiol Mol Biol Rev. 2004;68(3):560–602. [PMC free article: PMC515249] [PubMed: 15353570]
- Bychowska A, Theilacker C, Czerwicka M, Marszewska K, Huebner J, Holst O, et al. Chemical structure of wall teichoic acid isolated from Enterococcus faecium strain U0317. Carbohydr Res. 2011;346(17):2816–2819. [PubMed: 22024569]
- Caprioli T, Zaccour F, Kasatiya SS. Phage typing scheme for group D streptococci isolated from human urogenital tract. J Clin Microbiol. 1975;2(4):311–317. [PMC free article: PMC362801] [PubMed: 810495]
- Chhibber S, Kaur S, Kumari S. Therapeutic potential of bacteriophage in treating Klebsiella pneumoniae B5055-mediated lobar pneumonia in mice. J Med Microbiol. 2008;57(Pt 12):1508–1513. [PubMed: 19018021]
- Chibani-Chennoufi S, Dillmann ML, Marvin-Guy L, Rami-Shojaei S, Brüssow H. Lactobacillus plantarum bacteriophage LP65: a new member of the SPO1-like genus of the family Myoviridae. J Bacteriol. 2004;186(21):7069–7083. [PMC free article: PMC523202] [PubMed: 15489418]
- Clark PF, Clark AS. A bacteriophage active against a virulent hemolytic Streptococcus. Proc Soc Exp Biol Med. 1927;24(7):635–639.
- d’Hérouel AF, Wessner F, Halpern D, Ly-Vu D, Kennedy SP, Serror P, et al. A simple and efficient method to search for selected primary transcripts: non-coding and antisense RNAs in the human pathogen Enterococcus faecalis. Nucleic Acids Res. 2011;39(7):e46. [PMC free article: PMC3074167] [PubMed: 21266481]
- Deltcheva E, Chylinski K, Sharma CM, Gonzales K, Chao Y, Pirzada ZA, et al. CRISPR RNA maturation by trans-encoded small RNA and host factor RNase III. Nature. 2011;471:602–607. [PMC free article: PMC3070239] [PubMed: 21455174]
- DeMarini DM, Lawrence BK. Prophage induction by DNA topoisomerase II poisons and reactive-oxygen species: role of DNA breaks. Mutat Res. 1992;267(1):1–17. [PubMed: 1373845]
- Desiere F, Lucchini S, Canchaya C, Ventura M, Brüssow H. Comparative genomics of phages and prophages in lactic acid bacteria. Antonie van Leeuwenhoek. 2002;82(1-4):73–91. [PubMed: 12369206]
- Deutsch S-M, Guezenec S, Piot M, Foster S, Lortal S. Mur-LH, the broad-spectrum endolysin of Lactobacillus helveticus temperate bacteriophage phi-0303. Appl Environ Microbiol. 2004;70(1):96–103. [PMC free article: PMC321252] [PubMed: 14711630]
- Deveau H, Rodolphe B, Garneau J, Labonté J, Fremaux C, Boyaval P, et al. Phage response to CRISPR-encoded resistance in Streptococcus thermophilus. J Bacteriol. 2008;190(4):1390–1400. [PMC free article: PMC2238228] [PubMed: 18065545]
- Duerkop BA, Clements CV, Rollins D, Rodrigues JL, Hooper LV. A composite bacteriophage alters colonization by an intestinal commensal bacterium. Proc Natl Acad Sci U S A. 2012;109(43):17621–17626. [PMC free article: PMC3491505] [PubMed: 23045666]
- Evans AC. The Prevalence of Streptococcus Bacteriophage. Science. 1934;80(2063):40–41. [PubMed: 17818639]
- Fard RM, Barton MD, Arthur JL, Heuzenroeder MW. Whole-genome sequencing and gene mapping of a newly isolated lytic enterococcal bacteriophage EFRM31. Arch Virol. 2010;155(11):1887–1891. [PubMed: 20844906]
- Fischetti VA. Novel method to control pathogenic bacteria on human mucous membranes. Ann N Y Acad Sci. 2003;987:207–214. [PubMed: 12727641]
- Fischetti VA. Bacteriophage lytic enzymes: novel anti-infectives. Trends Microbiol. 2005;13(10):491–496. [PubMed: 16125935]
- Fischetti VA. Bacteriophage lysins as effective antibacterials. Curr Opin Microbiol. 2008;11(5):393–400. [PMC free article: PMC2597892] [PubMed: 18824123]
- Galloway-Peña J, Roh JH, Latorre M, Qin X, Murray BE. Genomic and SNP analyses demonstrate a distant separation of the hospital and community-associated clades of Enterococcus faecium. PLoS ONE. 2012;7(1):e30187. [PMC free article: PMC3266884] [PubMed: 22291916]
- Garneau JE, Dupuis M-È, Villion M, Romero DA, Barrangou R, Boyaval P, et al. The CRISPR/Cas bacterial immune system cleaves bacteriophage and plasmid DNA. Nature. 2010;468:67–71. [PubMed: 21048762]
- Grissa, I., Vergnaud, G., & Pourcel, C. (2007). CRISPRFinder: a web tool to identify clustered regularly interspaced short palindromic repeats. Nucleic Acids Research, 35(Web Server Issue), W52-W57. [PMC free article: PMC1933234] [PubMed: 17537822]
- Haft DH, Selengut J, Mongodin EF, Nelson KE. A guild of 45 CRISPR-associated (Cas) protein families and multiple CRISPR/Cas subtypes exist in prokaryotic genomes. PLOS Comput Biol. 2005;1(6):e60. [PMC free article: PMC1282333] [PubMed: 16292354]
- Hale CR, Zhao P, Olson S, Duff MO, Graveley BR, Wells L, et al. RNA-guided RNA cleavage by a CRISPR RNA-Cas protein complex. Cell. 2009;139(5):945–956. [PMC free article: PMC2951265] [PubMed: 19945378]
- Hancock LE, Gilmore MS. The capsular polysaccharide of Enterococcus faecalis and its relationship to other polysaccharides in the cell wall. Proc Natl Acad Sci U S A. 2002;99(3):1574–1579. [PMC free article: PMC122232] [PubMed: 11830672]
- Hatfull GF, Cresawn SG, Hendrix RW. Comparative genomics of the mycobacteriophages: insights into bacteriophage evolution. Res Microbiol. 2008;159(5):332–339. [PMC free article: PMC2632949] [PubMed: 18653319]
- Hendrix RW, Smith MC, Burns RN, Ford ME, Hatfull GF. Evolutionary relationships among diverse bacteriophages and prophages: all the world's a phage. Proc Natl Acad Sci U S A. 1999;96(5):2192–2197. [PMC free article: PMC26759] [PubMed: 10051617]
- Hogendorf WF, Bos LJ, Overkleeft HS, Codée JD, Marel GA. Synthesis of an alpha-kojibiosyl substituted glycerol teichoic acid hexamer. Bioorg Med Chem. 2010;18(11):3668–3678. [PubMed: 20409722]
- Horiuchi T, Sakka M, Hayashi A, Shimada T, Kimura T, Sakka K. Complete genome sequence of bacteriophage BC-611 specifically infecting Enterococcus faecalis strain NP-10011. J Virol. 2012;86(17):9538–9539. [PMC free article: PMC3416177] [PubMed: 22879611]
- Horvath P, Coûté-Monvoisin AC, Romero DA, Boyaval P, Fremaux C, Barrangou R. Comparative analysis of CRISPR loci in lactic acid bacteria genomes. Int J Food Microbiol. 2009;131(1):62–70. [PubMed: 18635282]
- Horvath P, Romero DA, Coûté-Monvoisin AC, Richards M, Deveau H, Moineau S, et al. Diversity, activity, and evolution of CRISPR loci in Streptococcus thermophilus. J Bacteriol. 2008;190(4):1401–1412. [PMC free article: PMC2238196] [PubMed: 18065539]
- Jansen R, Embden JD, Gasstra W, Schouls LM. Identification of genes that are associated with DNA repeats in prokaryotes. Mol Microbiol. 2002;43(6):1565–1575. [PubMed: 11952905]
- Johnson AD, Poteete AR, Lauer G, Sauer RT, Ackers GK, Ptashne M. λ Repressor and cro—components of an efficient molecular switch. Nature. 1981;294:217–223. [PubMed: 6457992]
- Kayaoglu G, Ørstavik D. Virulence factors of Enterococcus faecalis: relationship to endodontic disease. Crit Rev Oral Biol Med. 2004;15(5):308–320. [PubMed: 15470268]
- Krupovic M, Prangishvili D, Hendrix RW, Bamford DH. Genomics of bacterial and archaeal viruses: dynamics within the prokaryotic virosphere. Microbiol Mol Biol Rev. 2011;75(4):610–635. [PMC free article: PMC3232739] [PubMed: 22126996]
- Kunin V, Sorek R, Hugenholtz P. Evolutionary conservation of sequence and secondary structures in CRISPR repeats. Genome Biol. 2007;8:R61. [PMC free article: PMC1896005] [PubMed: 17442114]
- Labrie SJ, Samson JE, Moineau S. Bacteriophage resistance mechanisms. Nat Rev Microbiol. 2010;8(5):317–327. [PubMed: 20348932]
- LaTuga MS, Ellis JC, Cotton CM, Goldberg RN, Wynn JL, Jackson RB, et al. Beyond bacteria: a study of the enteric microbial consortium in extremely low birth weight infants. PLoS ONE. 2011;6(12):e27858. [PMC free article: PMC3234235] [PubMed: 22174751]
- Leavis HL, Willems RJ, van Wamel WJ, Schuren FH, Caspers MP, Bonten MJ. Insertion sequence-driven diversification creates a globally dispersed emerging multiresistant subspecies of E. faecium. PLoS Pathog. 2007;3(1):e7. [PMC free article: PMC1781477] [PubMed: 17257059]
- Lee SJ, Xie A, Jiang W, Etchegaray JP, Jones PG, Inouye M. Family of the major cold-shock protein, CspA (CS7.4), of Escherichia coli, whose members show a high sequence similarity with the eukaryotic Y-box binding proteins. Mol Microbiol. 1994;11(5):833–839. [PubMed: 8022261]
- Lee YD, Park JH. Complete genome sequence of enterococcal bacteriophage SAP6. J Virol. 2012;86(9):5402–5403. [PMC free article: PMC3347394] [PubMed: 22492926]
- Lepage E, Brinster S, Caron C, Ducroix-Crepy C, Rigottier-Gois L, Dunny G, et al. Comparative genomic hybridization analysis of Enterococcus faecalis: identification of genes absent from food strains. J Bacteriol. 2006;188(19):6858–6868. [PMC free article: PMC1595521] [PubMed: 16980489]
- Lindberg AA. Bacteriophage receptors. Annu Rev Microbiol. 1973;27:205–241. [PubMed: 4584686]
- Lindenstrauss AG, Pavlovic M, Bringmann A, Behr J, Ehrmann MA, Vogel RF. Comparison of genotypic and phenotypic cluster analyses of virulence determinants and possible role of CRISPR elements towards their incidence in Enterococcus faecalis and Enterococcus faecium. Syst Appl Microbiol. 2011;34(8):553–560. [PubMed: 21943678]
- Little JW, Mount DW. The SOS regulatory system of Escherichia coli. Cell. 1982;29(1):11–22. [PubMed: 7049397]
- Loeffler JM, Fischetti VA. Synergistic lethal effect of a combination of phage lytic enzymes with different activities on penicillin-sensitive and -resistant Streptococcus pneumoniae strains. Antimicrob Agents Chemother. 2003;47:375–377. [PMC free article: PMC149014] [PubMed: 12499217]
- Loeffler JM, Djurkovic S, Fischetti VA. Phage lytic enzyme Cpl-1 as a novel antimicrobial for pneumococcal bacteremia. Infect Immun. 2003;71(11):6199–6204. [PMC free article: PMC219578] [PubMed: 14573637]
- Makarova KS, Aravind L, Wolf YI, Koonin EV. Unification of Cas protein families and a simple scenario for the origin and evolution of CRISPR-Cas systems. Biol Direct. 2011;6:38. [PMC free article: PMC3150331] [PubMed: 21756346]
- Makarova KS, Grishin NV, Shabalina SA, Wolf YI, Koonin EV. A putative RNA-interference-based immune system in prokaryotes: computational analysis of the predicted enzymatic machinery, functional analogies with eukaryotic RNAi, and hypothetical mechanisms of action. Biol Direct. 2006;1:7. [PMC free article: PMC1462988] [PubMed: 16545108]
- Makarova KS, Haft DH, Barrangou R, Brouns SJ, Charpentier E, Horvath P, et al. Evolution and classification of the CRISPR-Cas systems. Nat Rev Microbiol. 2011;9(6):467–477. [PMC free article: PMC3380444] [PubMed: 21552286]
- Mandin P., Repoila F., Vergassola M.Geissmann, & Cossart, PIdentification of new noncoding RNAs in Listeria monocytogenes and prediction of mRNA targets Nucleic Acids Res 200735(3):962–974. [PMC free article: PMC1807966] [PubMed: 17259222]
- Manson JM, Hancock LE, Gilmore MS. Mechanism of chromosomal transfer of Enterococcus faecalis pathogenicity island, capsule, antimicrobial resistance, and other traits. Proc Natl Acad Sci U S A. 2010;107(27):12269–12274. [PMC free article: PMC2901427] [PubMed: 20566881]
- Marrafini LA, Sontheimer EJ. CRISPR interference limits horizontal gene transfer in staphylococci by targeting DNA. Science. 2008;322(5909):1843–1845. [PMC free article: PMC2695655] [PubMed: 19095942]
- Matos RC, Lapaque N, Rigottier-Gois L, Debarbieux L, Meylheuc T, Gonzalez-Zorn B, et al. Enterococcus faecalis prophage dynamics and contributions to pathogenic traits. PLoS Genet. 2013;9(6):e1003539. [PMC free article: PMC3675006] [PubMed: 23754962]
- Matsuzaki S, Yasuda M, Nishikawa H, Kuroda M, Ujihara T, Shuin T, et al. Experimental protection of mice against lethal Staphylococcus aureus infection by novel bacteriophage phi MR11. J Infect Dis. 2003;187(4):613–624. [PubMed: 12599078]
- Mazaheri Nezhad Fard R, Barton MD, Heuzenroeder MW. Novel Bacteriophages in Enterococcus spp. Curr Microbiol. 2010;60(6):400–406. [PubMed: 19967374]
- Mazaheri Nezhad Fard R, Barton MD, Heuzenroeder MW. Bacteriophage-mediated transduction of antibiotic resistance in enterococci. Lett Appl Microbiol. 2011;52(6):559–564. [PubMed: 21395627]
- McBride SM, Fischetti VA, Leblanc DJ, Moellering RC Jr, Gilmore MS. Genetic diversity among Enterococcus faecalis. PLoS ONE. 2007;2(7):e582. [PMC free article: PMC1899230] [PubMed: 17611618]
- McVay CS, Velásquez M, Fralick JA. Phage therapy of Pseudomonas aeruginosa infection in a mouse burn wound model. Antimicrob Agents Chemother. 2007;51(6):1934–1938. [PMC free article: PMC1891379] [PubMed: 17387151]
- Miller ES, Kutter KE, Mosiq G, Arisaka F, Kunisawa T, Rüger W. Bacteriophage T4 genome. Microbiol Mol Biol Rev. 2003;67(1):86–156. [table of contents.]. [PMC free article: PMC150520] [PubMed: 12626685]
- Minot S, Sinha R, Chen J, Li H, Keilbaugh SA, Wu GD, et al. The human gut virome: inter-individual variation and dynamic response to diet. Genome Res. 2011;21:1616–1625. [PMC free article: PMC3202279] [PubMed: 21880779]
- Mitchell J, Sullam PM. Streptococcus mitis phage-encoded adhesins mediate attachment to {alpha}2-8-linked sialic acid residues on platelet membrane gangliosides. Infect Immun. 2009;77(8):3485–3490. [PMC free article: PMC2715653] [PubMed: 19506011]
- Mojica FJ, Díez-Villaseñor C, García-Martínez J, Almendros C. Short motif sequences determine the targets of the prokaryotic CRISPR defence system. Microbiology. 2009;155(3):733–740. [PubMed: 19246744]
- Mojica FJ, Díez-Villaseñor C, García-Martínez J, Soria E. Intervening sequences of regularly spaced prokaryotic repeats derive from foreign genetic elements. J Mol Evol. 2005;60(2):174–182. [PubMed: 15791728]
- Mojica FJ, Díez-Villaseñor C, Soria E, Juez G. Biological significance of a family of regularly spaced repeats in the genomes of Archaea, Bacteria and mitochondria. Mol Microbiol. 2000;36(1):244–246. [PubMed: 10760181]
- Monk IR, Shah IM, Xu M, Tan MW, Foster TJ. Transforming the Untransformable: Application of Direct Transformation To Manipulate Genetically Staphylococcus aureus and Staphylococcus epidermidis. MBio. 2012;3(2):e00277–e00211. [PMC free article: PMC3312211] [PubMed: 22434850]
- Morowitz MJ, Denef VJ, Costello EK, Thomas BC, Poroyko V, Relman DA, et al. Strain-resolved community genomic analysis of gut microbial colonization in a premature infant. Proc Natl Acad Sci U S A. 2010;108:1128–1133. [PMC free article: PMC3024690] [PubMed: 21191099]
- Nallapareddy SR, Singh KV, Sillanpää J, Garsin DA, Höök M, Erlandsen SL, et al. Endocarditis and biofilm-associated pili of Enterococcus faecalis. J Clin Invest. 2006;116(10):2799–2807. [PMC free article: PMC1578622] [PubMed: 17016560]
- Nam KH, Kurinov I, Ke A. Crystal structure of clustered regularly interspaced short palindromic repeats (CRISPR)-associated Csn2 protein revealed Ca2+-dependent double-stranded DNA binding activity. J Biol Chem. 2011;286(35):30759–30768. [PMC free article: PMC3162437] [PubMed: 21697083]
- Nigutová K, Styriak I, Javorský P, Pristas P. Partial characterization of Enterococcus faecalis bacteriophage F4. Folia Microbiol (Praha). 2008;53(3):234–236. [PubMed: 18661299]
- Novick RP, Christie GE, Penadés JR. The phage-related chromosomal islands of Gram-positive bacteria. Nat Rev Microbiol. 2010;8(8):541–551. [PMC free article: PMC3522866] [PubMed: 20634809]
- Okhapkina SS, Netesova NA, Golikova LN, Seregina EV, Sosnovtsev SV, Abdurashitov MA, et al. Comparison of the homologous SfeI and LlaBI restriction-modification systems. J Mol Biol. 2002;36(3):432–437. [PubMed: 12068627]
- Osborn MJ. Structure and biosynthesis of the bacterial cell wall. Annu Rev Biochem. 1969;38:501–538. [PubMed: 4896244]
- Otawa K, Hirakata Y, Kaku M, Nakai Y. Bacteriophage control of vancomycin-resistant enterococci in cattle compost. J Appl Microbiol. 2012;113(3):499–507. [PubMed: 22702478]
- Paisano AF, Spira B, Cai S, Bombana AC. In vitro antimicrobial effect of bacteriophages on human dentin infected with Enterococcus faecalis ATCC 29212. Oral Microbiol Immunol. 2004;19(5):327–330. [PubMed: 15327646]
- Palmer KL, Gilmore MS. Multidrug-resistant enterococci lack CRISPR-cas. MBio. 2010;1(4):e00227–e00210. [PMC free article: PMC2975353] [PubMed: 21060735]
- Palmer KL, Carniol K, Manson JM, Heiman D, Shea T, Young S, et al. High-quality draft genome sequences of 28 Enterococcus sp. isolates. J Bacteriol. 2010;192(9):2469–2470. [PMC free article: PMC2863476] [PubMed: 20207762]
- Palmer KL, Daniel A, Hardy C, Silverman J, Gilmore MS. Genetic basis for daptomycin resistance in enterococci. Antimicrob Agents Chemother. 2011;55(7):3345–3356. [PMC free article: PMC3122436] [PubMed: 21502617]
- Palmer KL, Godfrey P, Griggs A, Kos VN, Zucker J, Desjardins C, et al. Comparative Genomics of Enterococci: Variation in Enterococcus faecalis, Clade Structure in E. faecium, and Defining Characteristics of E. gallinarum and E. casseliflavus. MBio. 2012;3(1):e00318–e00311. [PMC free article: PMC3374389] [PubMed: 22354958]
- Palmer KL, Kos VN, Gilmore MS. Horizontal gene transfer and the genomics of enterococcal antibiotic resistance. Curr Opin Microbiol. 2010;13(5):632–639. [PMC free article: PMC2955785] [PubMed: 20837397]
- Parasion S, Kwiatek M, Mizak L, Gryko R, Bartoszcze M, Kocik J. Isolation and characterization of a novel bacteriophage φ4D lytic against Enterococcus faecalis strains. Curr Microbiol. 2012;65(3):284–289. [PubMed: 22669253]
- Paulsen IT, Banerjei L, Myers GS, Nelson KE, Seshadri R, Read TD, et al. Role of mobile DNA in the evolution of vancomycin-resistant Enterococcus faecalis. Science. 2003;299(5615):2071–2074. [PubMed: 12663927]
- Pedulla ML, Ford ME, Houtz JM, Karthikeyan T, Wadsworth C, Lewis JA, et al. Origins of highly mosaic mycobacteriophage genomes. Cell. 2003;113(2):171–182. [PubMed: 12705866]
- Pourcel C, Salvignol G, Vergnaud G. CRISPR elements in Yersinia pestis acquire new repeats by preferential uptake of bacteriophage DNA, and provide additional tools for evolutionary studies. Microbiology. 2005;151(Pt 3):653–663. [PubMed: 15758212]
- Prestel E, Salamitou S, DuBow MS. An examination of the bacteriophages and bacteria of the Namib desert. J Microbiol. 2008;46(4):364–372. [PubMed: 18758725]
- Prévost G, Cribier B, Couppié P, Petiau P, Supersac G, Finck-Barbançon V, et al. Panton-Valentine leucocidin and gamma-hemolysin from Staphylococcus aureus ATCC 49775 are encoded by distinct genetic loci and have different biological activities. Infect Immun. 1995;63(10):4121–4129. [PMC free article: PMC173579] [PubMed: 7558328]
- Pride DT, Salzman J, Haynes M, Rohwer F, Davis-Long C, White RA 3rd, et al. Evidence of a robust resident bacteriophage population revealed through analysis of the human salivary virome. ISME J. 2012;6(5):915–926. [PMC free article: PMC3329113] [PubMed: 22158393]
- Prigent M, Leroy M, Confalonieri F, Dutertre M, DuBow MS. A diversity of bacteriophage forms and genomes can be isolated from the surface sands of the Sahara Desert. Extremophiles. 2005;9(4):289–296. [PubMed: 15947866]
- Proctor LM. The Human Microbiome Project in 2011 and beyond. Cell Host Microbe. 2011;10(4):287–291. [PubMed: 22018227]
- Ptashne M. (2004). A Genetic Switch: Phage Lambda Revisited (3rd ed.). Cold Spring Harbor, NY: Cold Spring Harbor Laboratory Press.
- Ptashne M, Jeffrey A, Johnson AD, Maurer R, Meyer BJ, Pabo CO, et al. How the lambda repressor and cro work. Cell. 1980;19(1):1–11. [PubMed: 6444544]
- Purnell SE, Ebdon JE, Tayor HD. Bacteriophage lysis of Enterococcus host strains: a tool for microbial source tracking? Environ Sci Technol. 2011;45(24):10699–10705. [PubMed: 22047499]
- Radlińska M, Piekarowicz A, Galimand M, Bujnicki JM. Cloning and preliminary characterization of a GATC-specific beta2-class DNA:m6A methyltransferase encoded by transposon Tn1549 from Enterococcus spp. Pol J Microbiol. 2005;54(3):249–252. [PubMed: 16450842]
- Räisänen L, Draing C, Pfitzenmaier M, Schubert K, Jaakonsaari T, von Aulock S, et al. Molecular interaction between lipoteichoic acids and Lactobacillus delbrueckii phages depends on D-alanyl and alpha-glucose substitution of poly(glycerophosphate) backbones. J Bacteriol. 2007;189(11):4135–4140. [PMC free article: PMC1913418] [PubMed: 17416656]
- Rakhuba DV, Kolomiets EI, Dey ES, Novik GI. Bacteriophage receptors, mechanisms of phage adsorption and penetration into host cell. Pol J Microbiol. 2010;59(3):145–155. [PubMed: 21033576]
- Rakieten ML, Tiffany EJ. The Absorption of Staphylococcus Bacteriophages by Enterococci. J Bacteriol. 1938;36(2):155–173. [PMC free article: PMC545339] [PubMed: 16560150]
- Reyes A, Haynes M, Hanson N, Angly FE, Heath AC, Rohwer F, et al. Viruses in the faecal microbiota of monozygotic twins and their mothers. Nature. 2010;466:334–338. [PMC free article: PMC2919852] [PubMed: 20631792]
- Roberts RJ. Restriction and modification enzymes and their recognition sequences. Nucleic Acids Res. 1984;12 Suppl:r167–r204. [PMC free article: PMC320008] [PubMed: 6328451]
- Roberts RJ, Vincze T, Posfai J, Macelis D. REBASE--a database for DNA restriction and modification: enzymes, genes and genomes. Nucleic Acids Res. 2010;38(Database issue):D234–D236. [PMC free article: PMC2808884] [PubMed: 19846593]
- Rogers CG, Sarles WB. Characterization of Enterococcus Bacteriophages from the Small Intestine of the Rat. J Bacteriol. 1963;85(6):1378–1385. [PMC free article: PMC278344] [PubMed: 14047233]
- Rousseau C, Gonnet M, Le Romancer M, Nicolas J. CRISPI: a CRISPR interactive database. Bioinformatics. 2009;25(24):3317–3318. [PMC free article: PMC2788928] [PubMed: 19846435]
- Santiago-Rodriguez TM, Dávila C, González J, Bonilla N, Marcos P, Urdaneta M, et al. Characterization of Enterococcus faecalis-infecting phages (enterophages) as markers of human fecal pollution in recreational waters. Water Res. 2010;44(16):4716–4725. [PubMed: 20723963]
- Sapranauskas R, Gasiunas G, Fremaux C, Barrangou R, Horvath P, Siksnys V. The Streptococcus thermophilus CRISPR/Cas system provides immunity in Escherichia coli. Nucleic Acids Res. 2011;39(21):9275–9282. [PMC free article: PMC3241640] [PubMed: 21813460]
- Sillanpää J, Prakash VP, Nallapareddy SR, Murray BE. Distribution of genes encoding MSCRAMMs and Pili in clinical and natural populations of Enterococcus faecium. J Clin Microbiol. 2009;47(4):896–901. [PMC free article: PMC2668297] [PubMed: 19193843]
- Solheim M, Brekke MC, Snipen LG, Willems RJ, Nes IF, Brede DA. Comparative genomic analysis reveals significant enrichment of mobile genetic elements and genes encoding surface structure-proteins in hospital-associated clonal complex 2 Enterococcus faecalis. BMC Microbiol. 2011;11:3. [PMC free article: PMC3022643] [PubMed: 21205308]
- Son JS, Jun SY, Kim EB, Park JE, Paik HR, Yoon SJ, et al. Complete genome sequence of a newly isolated lytic bacteriophage, EFAP-1 of Enterococcus faecalis, and antibacterial activity of its endolysin EFAL-1. J Appl Microbiol. 2010;108(5):1769–1779. [PubMed: 19863688]
- Srinivasiah S, Bhavsar J, Thapar K, Liles M, Schoenfeld T, Wommack KE. Phages across the biosphere: contrasts of viruses in soil and aquatic environments. Res Microbiol. 2008;159(5):349–357. [PubMed: 18565737]
- Stern A, Keren L, Wurtzel O, Amitai G, Sorek R. Self-targeting by CRISPR: gene regulation or autoimmunity? Trends Genet. 2010;26(8):335–340. [PMC free article: PMC2910793] [PubMed: 20598393]
- Stevens RH, Ektefaie MR, Fouts DE. The annotated complete DNA sequence of Enterococcus faecalis bacteriophage phiEf11 and its comparison with all available phage and predicted prophage genomes. FEMS Microbiol Lett. 2011;317(1):9–26. [PubMed: 21204936]
- Stevens RH, Porras OD, Delisle AL. Bacteriophages induced from lysogenic root canal isolates of Enterococcus faecalis. Oral Microbiol Immunol. 2009;24(4):278–284. [PubMed: 19572888]
- Stuart CH, Schwartz SA, Beeson TJ, Owatz CB. Enterococcus faecalis: its role in root canal treatment failure and current concepts in retreatment. J Endod. 2006;32(2):93–98. [PubMed: 16427453]
- Sugahara K, Yokoi KJ, Nakamura Y, Nishino T, Yamakawa A, Taketo A, et al. Mutational and biochemical analyses of the endolysin Lys(gaY) encoded by the Lactobacillus gasseri JCM 1131T phage phi gaY. Gene. 2007;404(1-2):41–52. [PubMed: 17920212]
- Tabor S, Richardson CC. A bacteriophage T7 RNA polymerase/promoter system for controlled exclusive expression of specific genes. Proc Natl Acad Sci U S A. 1985;82(4):1074–1078. [PMC free article: PMC397196] [PubMed: 3156376]
- Theilacker C, Kaczyński Z, Kropec A, Sava I, Bychowska A, Holst O, et al. Serodiversity of opsonic antibodies against Enterococcus faecalis--glycans of the cell wall revisited. PLoS ONE. 2011;6(3):e17839. [PMC free article: PMC3060912] [PubMed: 21437253]
- Thurlow LR, Thomas VC, Hancock LE. Capsular polysaccharide production in Enterococcus faecalis and contribution of CpsF to capsule serospecificity. J Bacteriol. 2009;191(20):6203–6210. [PMC free article: PMC2753019] [PubMed: 19684130]
- Tipper DJ, Strominger JL. Inhibition of cross-linking by penicillins and cephalosporins: studies in Staphylococcus aureus in vivo. J Biol Chem. 1968;243:3169–3179. [PubMed: 5653196]
- Tormo MÁ, Ferrer MD, Maiques E, Úbeda C, Selva L, Lasa I, et al. Staphylococcus aureus pathogenicity island DNA is packaged in particles composed of phage proteins. J Bacteriol. 2008;190(7):2434–2440. [PMC free article: PMC2293202] [PubMed: 18223072]
- Torres B, Jaenecke S, Timmis KN, García JL, Díaz E. A gene containment strategy based on a restriction-modification system. Environ Microbiol. 2000;2(5):555–563. [PubMed: 11233163]
- Uchiyama J, Rashel M, Maeda Y, Takemura I, Sugihara S, Akechi K, et al. Isolation and characterization of a novel Enterococcus faecalis bacteriophage phiEF24C as a therapeutic candidate. FEMS Microbiol Lett. 2008;278(2):200–206. [PubMed: 18096017]
- Uchiyama J, Rashel M, Takemura I, Wakiguchi H, Matsuzaki S. In silico and in vivo evaluation of bacteriophage phiEF24C, a candidate for treatment of Enterococcus faecalis infections. Appl Environ Microbiol. 2008;74(13):4149–4163. [PMC free article: PMC2446516] [PubMed: 18456848]
- Uchiyama J, Takemura I, Hayashi I, Matsuzaki S, Satoh M, Ujihara T, et al. Characterization of lytic enzyme open reading frame 9 (ORF9) derived from Enterococcus faecalis bacteriophage phiEF24C. Appl Environ Microbiol. 2011;77(2):580–585. [PMC free article: PMC3020548] [PubMed: 21097580]
- van Schaik W, Top J, Riley DR, Boekhorst J, Vrijenhoek JE, Schapendonk CM, et al. Pyrosequencing-based comparative genome analysis of the nosocomial pathogen Enterococcus faecium and identification of a large transferable pathogenicity island. BMC Genomics. 2010;11:239. [PMC free article: PMC2858755] [PubMed: 20398277]
- van Wamel WJ, Rooijakkers SH, Ruyken M, van Kessel KP, van Strijp JA. The innate immune modulators staphylococcal complement inhibitor and chemotaxis inhibitory protein of Staphylococcus aureus are located on beta-hemolysin-converting bacteriophages. J Bacteriol. 2006;188(4):1310–1315. [PMC free article: PMC1367213] [PubMed: 16452413]
- Villion M, Chopin MC, Deveau H, Ehrlich SD, Moineau S, Chopin A. P087, a lactococcal phage with a morphogenesis module similar to an Enterococcus faecalis prophage. Virology. 2009;388(1):49–56. [PubMed: 19349056]
- Wang IN, Smith DL, Young R. Holins: the protein clocks of bacteriophage infections. Annu Rev Microbiol. 2000;54:799–825. [PubMed: 11018145]
- Wang X, Kim Y, Ma Q, Hong SH, Pokusaeva K, Sturino JM, et al. Cryptic prophages help bacteria cope with adverse environments. Nat Commun. 2010;1:147. [PMC free article: PMC3105296] [PubMed: 21266997]
- Weinbauer MG. Ecology of prokaryotic viruses. FEMS Microbiol Rev. 2004;28(2):127–181. [PubMed: 15109783]
- Wiedenheft B, Lander GC, Zhou K, Jore MM, Brouns SJ, van der Oost J, et al. Structures of the RNA-guided surveillance complex from a bacterial immune system. Nature. 2011;477:486–489. [PMC free article: PMC4165517] [PubMed: 21938068]
- Wiedenheft B, Sternberg SH, Doudna JA. RNA-guided genetic silencing systems in bacteria and archaea. Nature. 2012;482:331–338. [PubMed: 22337052]
- Wiedenheft B, Zhou K, Jinek M, Coyle SM, Ma W, Doudna JA. Structural basis for DNase activity of a conserved protein implicated in CRISPR-mediated genome defense. Structure. 2009;17(6):904–912. [PubMed: 19523907]
- Willems RJ, Bonten MJ. Glycopeptide-resistant enterococci: deciphering virulence, resistance and epidemicity. Curr Opin Infect Dis. 2007;20(4):384–390. [PubMed: 17609597]
- Willner D, Furlan M, Haynes M, Schmeider R, Angly FE, Silva J, et al. Metagenomic analysis of respiratory tract DNA viral communities in cystic fibrosis and non-cystic fibrosis individuals. PLoS ONE. 2009;4(10):e7370. [PMC free article: PMC2756586] [PubMed: 19816605]
- Wolin MJ, Archibald AR, Baddiley J. Changes in wall teichoic acid resulting from mutations of Staphylococcus aureus. Nature. 1966;209:484–486. [PubMed: 5919578]
- Wommack KE, Colwell RR. Virioplankton: viruses in aquatic ecosystems. Microbiol Mol Biol Rev. 2000;64(1):69–114. [PMC free article: PMC98987] [PubMed: 10704475]
- Wu R, King CT, Jay E. A new sequence-specific endonuclease from Streptococcus faecalis subsp. zymogenes. Gene. 1978;4(4):329–336. [PubMed: 105969]
- Yasmin A, Kenny JG, Shankar J, Darby AC, Hall N, Edwards C, et al. Comparative genomics and transduction potential of Enterococcus faecalis temperate bacteriophages. J Bacteriol. 2010;192(4):1122–1130. [PMC free article: PMC2812964] [PubMed: 20008075]
- Yoong P, Schuch R, Nelson D, Fischetti VA. Identification of a broadly active phage lytic enzyme with lethal activity against antibiotic-resistant Enterococcus faecalis and Enterococcus faecium. J Bacteriol. 2004;186(14):4808–4812. [PMC free article: PMC438584] [PubMed: 15231813]
- Yosef I, Goren MG, Qimron U. Proteins and DNA elements essential for the CRISPR adaptation process in Escherichia coli. Nucleic Acids Res. 2012;40:5569–5576. [PMC free article: PMC3384332] [PubMed: 22402487]
- Young I, Wang I, Roof WD. Phages will out: strategies of host cell lysis. Trends Microbiol. 2000;8(3):120–128. [PubMed: 10707065]
- Young R. Bacteriophage lysis: mechanism and regulation. Microbiol Mol Biol Rev. 1992;56(3):430–481. [PMC free article: PMC372879] [PubMed: 1406491]
- Zhang J, Rouillon C, Kerou M, Reeks J, Brugger K, Graham S, et al. Structure and mechanism of the CMR complex for CRISPR-mediated antiviral immunity. Mol Cell. 2012;45(3):303–313. [PMC free article: PMC3381847] [PubMed: 22227115]
- Enterococcal Bacteriophages
- Distribution of phages across the enterococci
- Known phage families found among Enterococci
- Environments where enterococcal phages are found
- Enterococcal Temperate Phages and the Impact of Lysogeny
- Enterococcal Lytic Phages
- Enterococcal Genome Defense Mechanisms
- Perspectives and Future Directions
- Concluding Remarks
- References
- Review Tailed bacteriophages: the order caudovirales.[Adv Virus Res. 1998]Review Tailed bacteriophages: the order caudovirales.Ackermann HW. Adv Virus Res. 1998; 51:135-201.
- Toward Understanding Phage:Host Interactions in the Rumen; Complete Genome Sequences of Lytic Phages Infecting Rumen Bacteria.[Front Microbiol. 2017]Toward Understanding Phage:Host Interactions in the Rumen; Complete Genome Sequences of Lytic Phages Infecting Rumen Bacteria.Gilbert RA, Kelly WJ, Altermann E, Leahy SC, Minchin C, Ouwerkerk D, Klieve AV. Front Microbiol. 2017; 8:2340. Epub 2017 Dec 5.
- Novel Bacteriophages in Enterococcus spp.[Curr Microbiol. 2010]Novel Bacteriophages in Enterococcus spp.Mazaheri Nezhad Fard R, Barton MD, Heuzenroeder MW. Curr Microbiol. 2010 Jun; 60(6):400-6. Epub 2009 Dec 5.
- WGS analysis of two Staphylococcus aureus bacteriophages from sewage in China provides insights into the genetic feature of highly efficient lytic phages.[Microbiol Res. 2023]WGS analysis of two Staphylococcus aureus bacteriophages from sewage in China provides insights into the genetic feature of highly efficient lytic phages.Zhou WY, Wen H, Li YJ, Gao YJ, Zheng XF, Li HX, Zhu GQ, Zhang ZW, Yang ZQ. Microbiol Res. 2023 Jun; 271:127369. Epub 2023 Mar 24.
- Review Bacteriophages of lactobacilli.[Biochimie. 1988]Review Bacteriophages of lactobacilli.Sechaud L, Cluzel PJ, Rousseau M, Baumgartner A, Accolas JP. Biochimie. 1988 Mar; 70(3):401-10.
- Enterococcal Bacteriophages and Genome Defense - EnterococciEnterococcal Bacteriophages and Genome Defense - Enterococci
Your browsing activity is empty.
Activity recording is turned off.
See more...