The content of this book is licensed under a Creative Commons Attribution-NonCommercial-NoDerivs 4.0 Unported license. To view the terms and conditions of this license, visit https://creativecommons.org/licenses/by-nc-nd/4.0/
NCBI Bookshelf. A service of the National Library of Medicine, National Institutes of Health.
Varki A, Cummings RD, Esko JD, et al., editors. Essentials of Glycobiology [Internet]. 4th edition. Cold Spring Harbor (NY): Cold Spring Harbor Laboratory Press; 2022. doi: 10.1101/glycobiology.4e.53
Pure glycans of defined structure are essential research tools in glycobiology. Unlike proteins and nucleic acids, which can be obtained in homogeneous forms by recombinant expression and polymerase chain reaction (PCR), respectively, glycans produced in biological systems are heterogeneous. Furthermore, the quantities that can be obtained from biological systems are often small. Chemical synthesis can be used to obtain homogeneous glycans in larger quantities than are available from most cellular production systems. Chemical synthesis can be further employed to incorporate glycans into homogeneous glycoproteins. This chapter summarizes the current state of the art in chemical methods to produce glycans. Enzymes can be used together with chemical methods to prepare diverse glycans (Chapter 54).
CONTROLLING REGIOCHEMISTRY
The structure of oligosaccharides render their synthesis more complicated than the synthesis of the other major classes of biomolecules: oligonucleotides and oligopeptides. Fundamental challenges of glycan synthesis are the requirement for modifying one specific hydroxyl group in the presence of many others, and control over the stereochemical outcome in the creation of a glycosidic linkage. Commonly, glycan synthesis is characterized by the manipulation of various protecting groups, chemical moieties that mask the hydroxyl groups and prevent them from reacting with other chemical reagents. Hydroxyl-protecting groups are selectively added and removed from glycan structures, allowing for chemical alteration of the exposed hydroxyl groups. Subsequently, in a typical glycan synthesis scheme, the exposed hydroxyl group serves as a point for further elaboration. The selective exposure of one hydroxyl group allows for regioselective addition of another saccharide unit. Synthetic schemes of this type are commonly applied to the generation of O- and N-linked glycans (see Chapters 9 and 10) as well as proteoglycans (see Chapter 17) and glycosphingolipids (see Chapter 11).
The choice of protecting groups and the order of protecting group installation are essential for a successful synthetic route. The most common protecting groups include benzyl ethers that stay in place through many synthetic steps (permanent protecting groups) or carbonates and esters that are removed during intermediate steps in a synthesis (temporary protecting groups). A wealth of information exists on the chemical generation of glycans and various protecting group manipulations used in the context of glycan synthesis.
CONTROLLING STEREOCHEMISTRY
A glycosidic linkage is generally formed through the activation of a glycosylating agent (glycosyl donor) to create an electrophilic species that reacts with the nucleophile, for example, a hydroxyl group present on the glycosyl acceptor. Other possible acceptors include serine/threonine in the case of glycopeptides or a sphingoid in the case of glycosphingolipids. The glycosylation reaction results in the formation of either an α- or β-glycosidic linkage (see Chapter 2). A major challenge in glycan synthesis is the stereoselective formation of glycosidic bonds (Figure 53.1A; Chapter 2). A variety of methods are available to stereoselectively generate glycosidic linkages. The yield and the stereochemical outcome of these reactions depend on the steric and electronic nature of the glycosylating agent (the glycosyl donor), the nature of the nucleophile, and the reaction conditions. One common method to control the stereochemistry at the anomeric center involves the use of certain protecting groups, such as esters or amides/carbamates, on the C2-hydroxyl or C2-amino group (Figure 53.1B). These “participating neighboring” protecting groups can form a cyclic oxonium ion intermediate during the glycosylation reaction that shields one face of the molecule, leading exclusively to the formation of a “trans”-glycosidic linkage (i.e., where the anomeric substituent and the C2 group are on opposite sides of the ring, as in β-glucosides). The opposite anomeric stereochemistry, termed a “cis”-glycosidic bond, is more difficult to construct with high selectivity. Many different synthetic procedures have been developed for the stereoselective construction of cis-glycosidic linkages and linkages involving C2-deoxy sugars, though not as general as protecting group participation in the synthesis of trans-glycosidic linkages. The use of particular solvents/additives, remote protecting group participation, or preorganization of donor and acceptor and other methods have allowed for the stereocontrolled formation of an increasing number of cis-glycosidic linkages.

FIGURE 53.1.
(A) Stereospecific formation of glycosidic bonds as either an α- or β-linkage. (LG) Leaving group, (R) protecting group. (B) Formation of a cyclic oxonium ion intermediate leading to the formation of a β-glycosidic linkage.
PROTECTIVE GROUP MANIPULATIONS
Carbohydrates are endowed with a wealth of functional groups. This complicates glycan synthesis, and considerable time and effort are devoted to the development of suitable protective group schemes that allow for the protection and deprotection of individual functional groups at will. Carbohydrate functional groups include hydroxyls, amines, and carboxylates, and distinguishing between these is relatively easy. Selective address of hydroxyls—the most abundant functional groups in carbohydrates—is somewhat more complicated. Primary hydroxyls and anomeric hydroxyls are relatively easy to manipulate—specifically, the former for steric reasons and the latter because they are part of a hemiacetal or hemiketal. Specific access to secondary hydroxyls requires chemistry tailored to capitalize on, for instance, their configuration (cis-hydroxyls vs. trans-hydroxyls, equatorial vs. axial) and their relative reactivity (i.e., exploiting steric and/or electronic differences). A representative protective group scheme starting from S-tolylglucopyranose (the S-tolyl group serves as both a masking group of the anomeric hydroxyl and as leaving group in glycosylation schemes) is depicted in Figure 53.2. In the first step, all hydroxyls are transformed into the corresponding trimethylsilyl ethers to render the polar carbohydrate soluble in organic solvents. Next and in a series of complementary functional group manipulations, either O-2 (A), O-4 (B), or O-6 (C) is selectively liberated. In all instances, the first step comprises creation of the 4,6-O-benzylidene species. Reductive benzylation of O-3 followed by benzoylation of O-2 and reductive opening of the benzylidene toward the O-4-benzyl delivers glucose building block C. Alternative reductive opening of the benzylidene toward the O-6-benzyl delivers B, whereas leaving the benzylidene intact and removal of any remaining silyl protective groups delivers glucoside A.

FIGURE 53.2.
Protective group manipulations, which can be carried out in one-pot procedures, toward a series of glucopyranose-derived building blocks for glycan assembly.
A REPRESENTATIVE SOLUTION PHASE CHEMICAL GLYCAN SYNTHESIS
In the synthesis of a fragment of a putative repeating unit of the exopolysaccharide of Pseudomonas aeruginosa (Figure 53.3) the interplay between protective group pattern and glycosylation strategies becomes apparent. Glycosylation of 4,6-O-benzylidene protected thiomannoside 1 with orthogonally protected fucoside 2 promoted by benzenesulfinylpiperidine (BSP) and triflic anhydride (Tf2O) affords disaccharide 3. The stereoselective creation of the β-mannosidic linkage follows a SN2 displacement of an intermediate anomeric α-triflate stabilized by the benzylidene moiety.

FIGURE 53.3.
Solution phase synthesis of Pseudomonas aeruginosa–derived decasaccharide 10.
Oxidative removal of the naphthyl (Nap) protecting group and glycosylation with mannoside 4 gives trisaccharide 5. The anomeric allyl (All) group on the reducing fucoside is removed and transformed into the glycosyl trichloroacetimidate, which is condensed with the 3-hydroxyl group of orthogonally protected glucoside 6 to give tetrasaccharide 7. A 2-O-benzoyl (Bz) participating group in fucose building block 2 ensures the 1,2-trans-configuration. Elaboration of tetrasaccharide 7 led to fully protected decasaccharide 10 and illustrates the need for the two differently protected building blocks 1 and 4. The benzyl (Bn) protective protecting group in 1 serves as a permanent protecting group destined for removal at the final stages of the synthesis. In contrast, The tertbutyldimethylsilyl (TBS) group in 4 can be removed selectively during the synthesis. The 2-hydroxyl groups in mannose residues are liberated and 1,2-trans (α) mannosylated with building block 8. Global deprotection of 9 is effected in two steps: base treatment to remove acyl protective groups followed by catalytic hydrogenation to simultaneously remove benzyl, naphthyl, and benzylidene groups. The azide at the reducing end spacer is transformed into the amine for neoglycoprotein synthesis for immunization studies, or to produce glycan arrays.
REPRESENTATIVE AUTOMATED GLYCAN ASSEMBLY
Automated solid-phase synthesis has made great impact on peptide/protein and nucleic acid chemistry and biology. This development in synthetic chemistry made peptides and oligonucleotides available to biologists and facilitated studies of biopolymer structure and function. Likewise, the availability of a general automated (solid-phase) method for glycan synthesis vastly accelerates access to homogeneous material for biological studies. Because oligosaccharide synthesis is much more complex than the assembly of oligopeptides and nucleotides, automated procedures for glycan assembly were slower to evolve, but automated synthesizers are now commercially available. A prerequisite in automated glycan assembly (AGA) is full control over the stereochemistry of a newly formed glycosidic linkage. AGA is based on monosaccharide building blocks with orthogonal protecting groups and a linker. Important added value of AGA is the ability to rapidly generate libraries of related but distinct glycans, and the ability to prepare polysaccharides of a size not easily accessible with other methods.
The synthesis of a 100-mer polymannoside commences with placing a polystyrene Merrifield resin equipped with a photo-cleavable linker 11 in the reaction vessel of an automated synthesizer. Mannose thioglycoside building block 12 is employed for AGA using a four-step cycle (Figure 53.4A). The incorporation of each monomer relies on an acidic washing step to prevent quenching by any remaining base, followed by the coupling step using 5-6.5 equivalents of building block 12. Next, a capping step prevents any unreacted nucleophiles from engaging in subsequent couplings to produce (n-1)-polysaccharides that are difficult to separate from the desired product. Removal of the temporary Fmoc protecting group exposes the nucleophile for the subsequent coupling step. The 100 coupling cycles were executed automatically within 188 hours and yielded 100-mer α-(1-6) polymannoside 13 after photo-cleavage from the resin and normal-phase HPLC purification (8% overall yield). Cleavage of all ester protective groups by treatment with sodium methoxide, followed by palladium catalyzed hydrogenolysis under pressure to release all benzyl ether protective groups produced 100-mer polymannoside 14 after preparative reverse-phase HPLC.
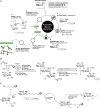
FIGURE 53.4.
(A) Schematic overview of automated glycan assembly. (B) Automated glycan assembly (AGA) of 100-polymannoside 14. (C) AGA of an alginate dodecasaccharide.
The synthesis of an alginate dodecamer composed of the β-1,4-mannuronate moieties is of note because it required the repetitive installation of cis-glycosidic linkages. The synthesis makes use of immobilized allyl alcohol 15. Reaction with donor mannuronic ester 16 under catalytic amounts of triflic acid (TfOH) yields immobilized monosaccharide 17. Removal of the levulinoyl (Lev) group at O-4 is accomplished by treatment with hydrazine (H2N-NH2) monoacetate, after which the coupling-deprotection sequence is repeated for the desired number of times. Upon completion of the glycan assembly, the fully protected oligosaccharide is removed from the solid support by cross metathesis and subsequent removal of all protecting groups by treatment with strong base and hydrogenolysis.
More complex polysaccharides are accessible by block couplings of polysaccharides prepared by AGA. Two polysaccharide blocks, linear 30-mer α-(1-6) polymannoside 21 prepared by AGA from mannose building block 12 and branched 31-mer polymannoside 23 prepared by AGA using 12 and differentially protected mannose building block 22 for the branching positions were unified in a 31+30+30+30+30 coupling to create branched 151-mer 24 that was deprotected to furnish 25 (Figure 53.5).

FIGURE 53.5.
Synthesis of a 151-mer polymannoside by block coupling of polymannosides prepared by automated glycan assembly (AGA).
COMPUTER-ASSISTED GLYCAN ASSEMBLY
A database of relative reactivity values (RRVs a number indicating how reactive a glycosyl donor is) of thioglycoside donors has been used to design one-pot glycosylation sequences. Several thioglycosides are combined and selective activation of one of the building blocks over the others can be achieved. The Optimer computer program aids in the selection of the combination of building blocks, equipped with the proper set of protecting groups that tune the reactivity. Figure 53.6 shows the one-pot assembly of the tumor associated N3 antigen octasaccharide using the Optimer approach. Three building blocks were designed and synthesized: fucosyl donor 26 (RRV = 7.2 × 104), lactosamine donor 27 (RRV = 41), and lactose building block 28 (RRV = 0). The latter disaccharides were prepared by chemoselective glycosylations exploiting RRVs. Combination of the first two building blocks in a BSP/Tf2O mediated condensation gave the trisaccharide thioglycoside. Addition of lactose 28 to the reaction vessel and NIS/TfOH to promote the ensuing double glycosylation provided the octasaccharide 29. After deprotection the spacer functionalized octasaccharide 30 was obtained in 11% yield.
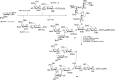
FIGURE 53.6.
One-pot synthesis of octasaccharide antigen 32.
OUTLOOK
Over the past quarter century, the chemical synthesis of glycans has advanced from an art practiced by synthetic chemists that engaged in heroic total synthesis efforts to a routine task for glycans that contain common monomeric constituents and trans-glycosidic linkages. With the commercialization of AGA in which a set of common building blocks is combined on a solid phase resin, the synthesis process can be greatly accelerated. AGA has become an important part of carbohydrate synthesis as polysaccharides as long as 100-mers as well as glycosaminoglycans are now rapidly accessible in multimilligram quantities. The production of highly branched structures will become routine in the coming years. Still, for challenging targets, containing rare monosaccharides and 2-deoxy and 1,2-cis-linkages, improved methods for solution phase synthesis will have to be developed. These methods will greatly benefit from the advances in fundamental knowledge on the glycosylation reaction and will be aided by knowledge gathered in databases. In addition, enzymatic synthesis and combinations of chemical and enzymatic synthesis are important assets to procure large quantities of glycans.
ACKNOWLEDGMENTS
The authors acknowledge contributions to previous versions of this chapter by Nathaniel Finney and David Rabuka and appreciate helpful comments and suggestions from Martina Delbianco.
FURTHER READING
- Plante OJ, Palmacci ER, Seeberger PH. 2001. Automated solid-phase synthesis of oligosaccharides. Science 291: 1523–1527. doi:10.1126/science.1057324 [PubMed: 11222853] [CrossRef]
- Wang CC, Lee JC, Luo SY, Kulkarni SS, Huang YW, Lee YW, Chang KL, Hung SC. 2007. Regioselective one-pot protection of carbohydrates. Nature 446: 896–899. doi:10.1038/nature05730 [PubMed: 17443183] [CrossRef]
- Zhu X, Schmidt RR. 2009. New principles for glycoside-bond formation. Angew Chem Int Ed 48: 1900–1934. doi:10.1002/anie.200802036 [PubMed: 19173361] [CrossRef]
- Crich D. 2010. Mechanism of a chemical glycosylation reaction. Acc Chem Res 43: 1144–1153. doi: 10.1021/ar100035r [PubMed: 20496888] [CrossRef]
- Codée JDC, Ali A, Overkleeft HS, van der Marel GA. 2011. Novel protecting groups in carbohydrate chemistry. C R Chimie 14: 178–193. doi:10.1016/j.crci.2010.05.010 [CrossRef]
- Hsu CH, Hung SC, Wu CY, Wong CH. 2011. Toward automated oligosaccharide synthesis. Angew Chem Int Ed 50: 11872–11923. doi:10.1002/anie.201100125 [PubMed: 22127846] [CrossRef]
- Walvoort MTC, van den Elst H, Plante OJ, Kröck L, Seeberger PH, Overkleeft HS, van der Marel GA, Codée JDC. 2012. Automated solid-phase synthesis of β-mannuronic acid alginates. Angew Chem Int Ed 51: 4393–4396. doi:10.1002/anie.201108744 [PubMed: 22334421] [CrossRef]
- Li H, Mo KF, Wang Q, Stover CK, DiGiandomenico A, Boons GJ. 2013. Epitope mapping of monoclonal antibodies using synthetic oligosaccharides uncovers novel aspects of immune recognition of the Psl exopolysaccharide of Pseudomonas aeruginosa. Chem Eur J 19: 17425–17431. doi:10.1002/chem.201302916 [PubMed: 24248772] [CrossRef]
- Hahm HS, Schlegel MK, Hurevich M, Eller S, Schuhmacher F, Hofmann J, Pagel K, Seeberger PH. 2017. Automated glycan assembly using the Glyconeer 2.1® synthesizer. Proc Nat Acad Sci 114: E3385–E3389. doi:10.1073/pnas.1700141114 [PMC free article: PMC5410834] [PubMed: 28396442] [CrossRef]
- Guberman M, Seeberger PH. 2019. Automated glycan assembly: a perspective. J Am Chem Soc 141: 5581–5592. doi:10.1021/jacs.9b00638 [PMC free article: PMC6727384] [PubMed: 30888803] [CrossRef]
- Joseph A, Pardo-Vargas A, Seeberger PH. 2020. Total synthesis of polysaccharides by automated glycan assembly. J Am Chem Soc 142: 8561–8564. doi:10.1021/jacs.0c00751 [PMC free article: PMC7304863] [PubMed: 32338884] [CrossRef]
- Review Chemical Synthesis of Glycans and Glycoconjugates.[Essentials of Glycobiology. 2015]Review Chemical Synthesis of Glycans and Glycoconjugates.Seeberger PH, Overkleeft HS. Essentials of Glycobiology. 2015
- Review Chemical and Enzymatic Synthesis of Glycans and Glycoconjugates.[Essentials of Glycobiology. 2009]Review Chemical and Enzymatic Synthesis of Glycans and Glycoconjugates.Seeberger PH, Finney N, Rabuka D, Bertozzi CR. Essentials of Glycobiology. 2009
- Review Chemoenzymatic Synthesis of Glycans and Glycoconjugates.[Essentials of Glycobiology. 2022]Review Chemoenzymatic Synthesis of Glycans and Glycoconjugates.Overkleeft HS, Seeberger PH. Essentials of Glycobiology. 2022
- Review Chemoenzymatic Synthesis of Glycans and Glycoconjugates.[Essentials of Glycobiology. 2015]Review Chemoenzymatic Synthesis of Glycans and Glycoconjugates.Overkleeft HS, Seeberger PH. Essentials of Glycobiology. 2015
- Chemical approaches to perturb, profile, and perceive glycans.[Acc Chem Res. 2009]Chemical approaches to perturb, profile, and perceive glycans.Agard NJ, Bertozzi CR. Acc Chem Res. 2009 Jun 16; 42(6):788-97.
- Chemical Synthesis of Glycans and Glycoconjugates - Essentials of GlycobiologyChemical Synthesis of Glycans and Glycoconjugates - Essentials of Glycobiology
Your browsing activity is empty.
Activity recording is turned off.
See more...